Introduction
Environmental problems caused by hazardous wastes and toxic water pollutants produced from textile, paper, plastic, and other industries have attracted considerable attention, because of the extensive use of organic dyes in the corresponding industrial processes.1-4 The physical, chemical, and biological methods have been developed for reducing the amount of pollutants in the environment.5,6 However, these approaches proved ineffective because the pollutants are not broken down but merely converted to other species, leading to secondary pollution.7 In recent years, great concern has focused on semiconductor photocatalysts owing to their potential application for the treatment of environmental pollutants.1,2,8
Different from traditional techniques, photocatalysis is one of the advanced oxidation processes that can convert pollutants into safer products such as CO2, H2O, and mineral acids.9,10 In particular, photocatalysis is based on electron-hole pairs generated in the semiconductor materials.11 The small size and high optical activity of semiconductors favor their use in the degradation of compounds appeared in the environment. When a semiconductor material absorbs higher photon energy than its band-gap energy, excited electrons and holes are generated. The generation of electrons and holes is enhanced in nanoscale semiconductors, due to the photon absorption and high electron density of active surface states associated with high ratio of their surface to volume. The generated electron-hole pairs lead to the formation of free radicals, which can undergo redox reactions with the compounds adsorbed on the surface of the photocatalyst.12
Semiconductor materials have been employed in a number of applications including optical coatings, optical sensors, photoconductors, photocatalysts, and other light-emitting systems.13 Various semiconductor materials based on zinc oxide and titanium dioxide have been investigated for the photocatalytic degradation of organic compounds.14,15 However, a limited number of studies have focused on zinc sulfide, because of some intrinsic limitations.2 Zinc sulfide has a relatively wide band gap of 3.6 eV and is generally used as an optoelectronic device material in the violet and blue regions.13 As a nontoxic semiconductor, zinc sulfide also has a higher chemical stability than other semiconductor materials.16
On average, doped semiconductor nanomaterials have a superior transition probability and electronic energy structure to their undoped counterparts.17By doping semiconductor materials with magnetic elements, the doped materials can also acquire magnetic and magneto-optical properties.13 Previous studies have reported doping of zinc sulfide nanoparticles with both rare earth metal (e.g., Eu) and transition metal (e.g., Cu, Ni, and Mn) elements.18-21 Among these nanomaterials, Mn-doped ZnS is the most extensively investigated, because of its high effective luminescence.22 Moreover, Mn-doped ZnS nanomaterials exhibit unique magneto-optical performances and an increased number of optically active sites.23
Zinc sulfide doped with Mn2+ ions is usually prepared by chemical precipitation, mechanochemical, sol-gel, colloidal solution, ultrasonic radiation, coating, and spray-based methods.24-29 Functional groups such as carboxyl and amino groups act as nucleation sites in the synthesis process.28 In this study, ZnS:Mn-glycine nanocomposites were synthesized using zinc sulfate heptahydrate (ZnSO4·7H2O), glycine (C2H5NO2), manganese sulfate monohydrate (MnSO4·H2O), and sodium sulfide (Na2S) precursors. ZnS:Mn-Gly-C60 nanocomposites were obtained by heating the ZnS:Mn-Gly nanocomposite and fullerene (C60) at a 2:1 mass ratio in an electric furnace at 700°C for 2 h. The ZnS:Mn-Gly-C60 nanocomposites were used as photocatalyst and we evaluated their photocatalytic activity for the degradation of brilliant green (BG) under 254 nm irradiation.
Experimental
Zinc sulfate heptahydrate, manganese sulfate monohydrate, ethanol and tetrahydrofuran (THF) were obtained from Samchun Pure Chemical Co., Ltd. Glycine was obtained from Duksan Pharmaceutical Co., Ltd. Sodium sulfide hydrate (Na2S·xH2O) and brilliant green (BG) were supplied by Sigma-Aldrich. Fullerene (C60) was purchased from Tokyo Chemical Industry Co., Ltd.
All chemicals were used as received without any further purification or treatment. Pure distilled water was used in all synthetic steps.
The ZnS:Mn-Gly nanocomposites were synthesized in a typical synthetic method, 50 mL of 0.1 M zinc sulfate solution was added dropwise to a 50 mL of 0.2 M glycine solution obtained by stirring in an ice-water bath for 1 h. The mixed solution was placed in a three-necked flask and deoxygenated in a nitrogen atmosphere for 30 min. Then, 20 mL of an aqueous solution containing 0.1 mmol of manganese sulfate and 5 mmol of sodium sulfide was rapidly injected into the solution under vigorous stirring condition. The final solution was refluxed for 10 h and the precipitated ZnS:Mn-Gly nanocomposites were washed five times with an ethanol-water solution and separated by centrifugation. To obtain powder sample, the centrifuged ZnS:Mn-Gly nanocomposites were dried for 10 h in an oven at 100°C. To synthesize ZnS:Mn-Gly-C60 nanocomposites, the ZnS:Mn-Gly nanocomposite and fullerene (C60) in a 2:1 mass ratio were stirred in 10 mL of THF for 30 min. Then, the mixture was placed in a hood and THF was removed by exposing the mixture to air. Subsequently, the mixture of ZnS:Mn-Gly/fullerene (C60) was calcined in an electric furnace (Ajeon Heating Industry Co., Ltd.) at 700°C for 2 h to obtain the ZnS:Mn-Gly-C60 nanocomposites.
The crystal structure of the ZnS:Mn-Gly and ZnS:Mn-Gly-C60 nanocomposites were characterized by X-ray powder diffraction (XRD) using Cu Kα radiation (Bruker, D8 Advance, λ = 1.54178 Å). The photocatalytic properties of the nanocomposites were characterized by UV-vis spectroscopy, using a Shimadzu UV-1619PC spectrophotometer in the range of 200-800 nm.
The photocatalytic degradation of brilliant green (BG) was investigated by placing 10 mL of organic dye solution, with an absorbance value of 1.0, in a 10 mL glass vial containing 500 mg/L of ZnS:Mn-Gly-C60 nanocomposites as photocatalyst. As a reactor, the glass vial was kept in a dark environment for 30 min to achieve adsorption-desorption equilibrium between organic dye and photocatalyst. Then, the vial was irradiated with a UV lamp (8 W, 254 nm, 77202 Marne-la-Vallée-cedex 1, France) set at a distance of 1 cm from the reactor. The photocatalytic degradation of brilliant green was monitored by the UV-vis spectra at fixed intervals.
Results and Discussion
Figure 1(a) shows the X-ray diffraction pattern of ZnS:Mn-Gly nanocomposites. The observed broad peaks, a typical feature of nanocrystalline samples, reveal that the sample has a zinc blende crystal structure. The diffraction peaks at 2θ = 28.6°, 47.9°, and 56.8° correspond to the (111), (220), and (311) planes, respectively, of the cubic structure of ZnS (ICDD PDF No. 65-0309).11,30,31 The XRD pattern of the ZnS:Mn-Gly-C60 nanocomposites are shown in Figure 1(b). Except for the peaks at 2θ = 28.7°, 47.7°, and 56.6° corresponding to the (111), (220), and (311) planes of ZnS, respectively, the peaks at 2θ = 10.9°, 17.8°, 20.9°, 21.8°, and 27.2° match perfectly with the (111), (220), (311), (222), and (420) crystalline planes of C60 powder, respectively (JCPDS card No. 44-0558).32 The XRD patterns of the ZnS:Mn-Gly and the ZnS:Mn-Gly-C60 nanocomposites show that the latter nanocomposites have a higher crystallinity than ZnS:Mn-Gly nanocomposites.
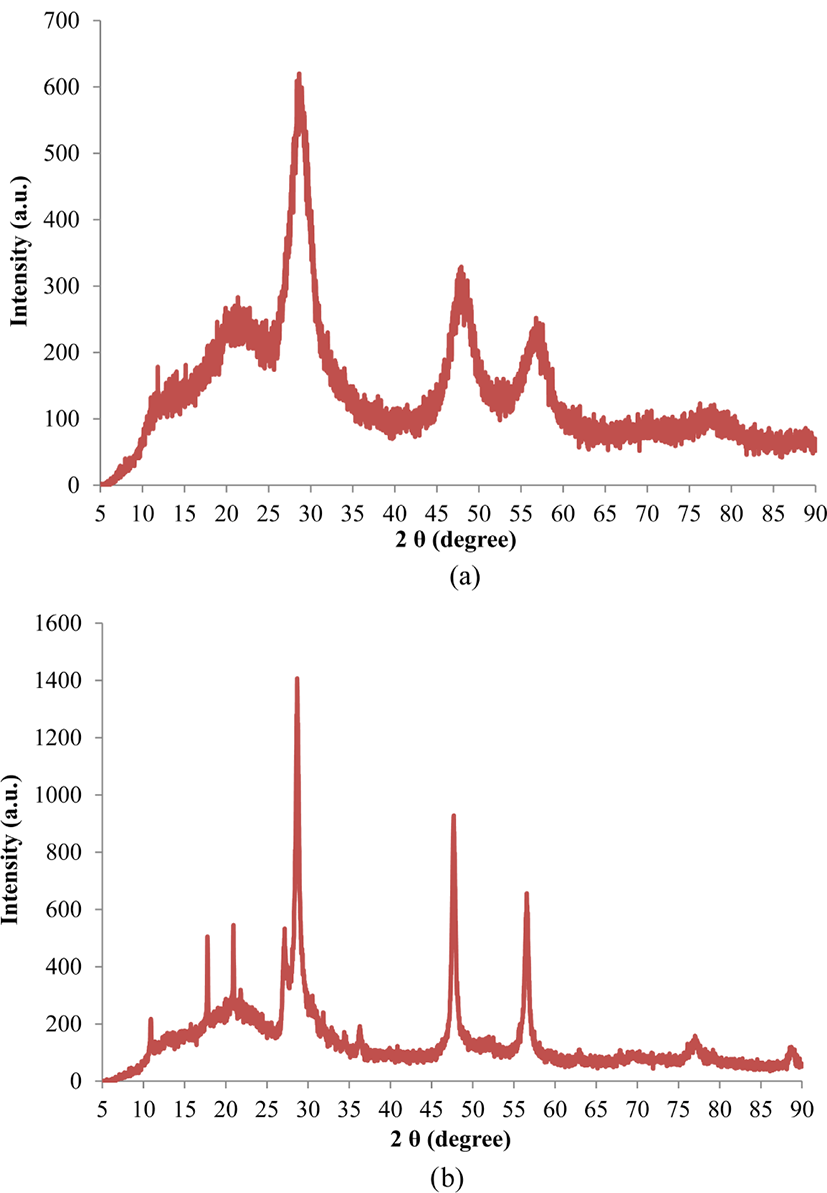
Figure 2 illustrates the degradation progress of brilliant green (BG) in the presence of ZnS:Mn-Gly-C60 nanocompo-site photocatalyst, under 254 nm irradiation at the time interval of 30 min.
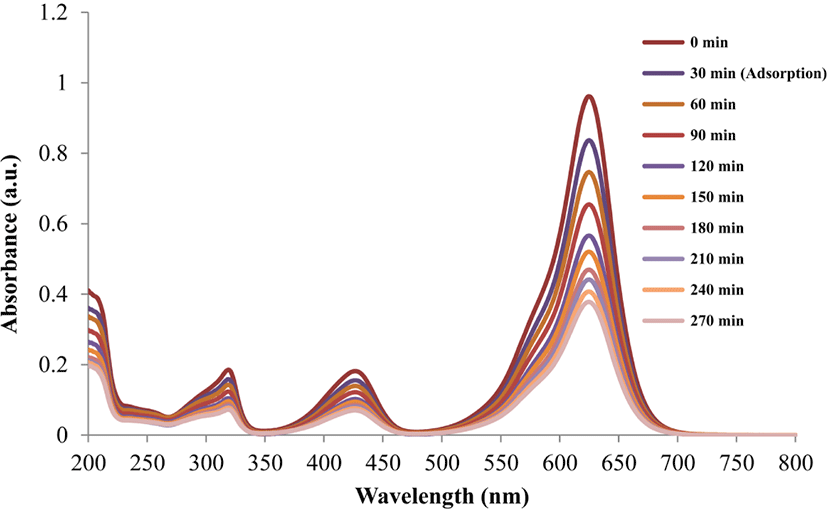
The absorbance value of λmax in brilliant green decreased with increasing irradiation time, due to the degradation of the dye.
To further investigate the photocatalytic efficiency of the nanocomposites, the kinetics of the photocatalytic degradation was studied using the Langmuir-Hinshelwood model.11 The reaction rate constant was calculated according to the following equation:
where t is the irradiation time and K is the rate constant of the reaction, which can thus be calculated from the slope of the plot.
Figure 3 shows a pseudo first order reaction in the photocatalytic degradation of BG by the ZnS:Mn-Gly-C60 nanocomposites. The reaction rate constant for photocatalytic degradation of BG by the ZnS:Mn-Gly-C60 nano-composite was calculated as a 3.3×10−3.
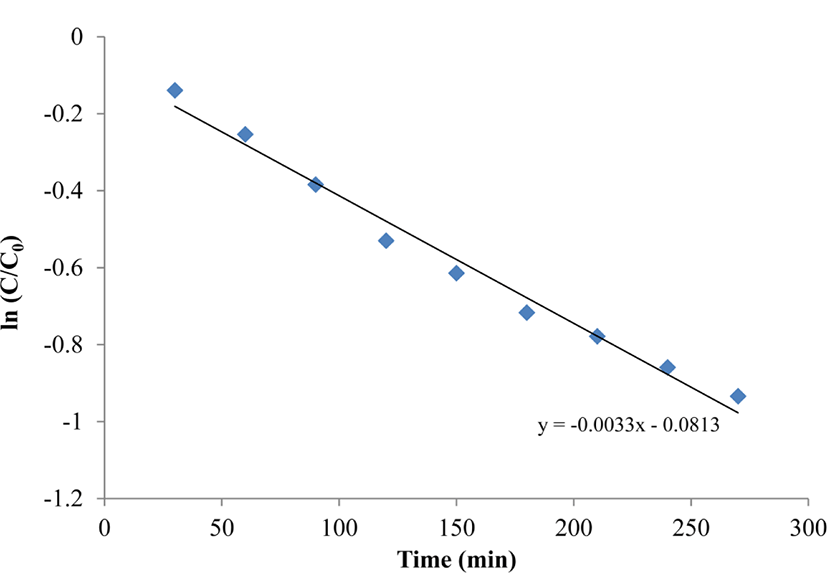
Conclusions
In the present study, ZnS:Mn-Gly-C60 nanocomposites were prepared by capping glycine on ZnS:Mn nanocomposites and fullerence (C60), followed by a calcination process. The structural property of the sample was characterized by X-ray diffraction. The photocatalytic activity of the ZnS:Mn-Gly-C60 nanocomposites was investigated by monitoring the degradation of brilliant green under ultraviolet irradiation. The photocatalytic degradation of BG using on ZnS:Mn-Gly-C60 nanocomposites followed a pseudo first order reaction rate law.