Introduction
Superabsorbent hydrogels (SAHs) are crosslinked hydrophilic polymers by crosslinker comprising a flexible polymer chain structure that can absorb, swell, and retain aqueous solutions of up to a hundred times of their own dry weight. Because these polymers are chemically or physically crosslinked, they swell to their equilibrium volume on the introduction of a large amount of water instead of dissolving in it.1-3
A hydrogel is a gel that contains water as a dispersion medium. The hydrosol loses fluidity due to the cooling or the hydrophilic polymer having a three-dimensional network structure. The microcrystalline structure expands and forms a hydrogel containing water. The hydrogels of the electrolytic polymer are highly water-absorbent and are widely used in many fields such as those involving agriculture, chemical sensors, disposable personal hygiene products, cosmetics, food, controlled drug delivery systems, and wound dressings.4-12
The ideal characteristics of a good SAH for practical applications include a high water absorbency (it should absorb and retain large amounts of water under a load), high gel strength, high liquid permeability, and fast absorption. During swelling, the polymer should be able to absorb and desorb fluid multiple times without degradation (in some uses, the fluid absorption should be reversible). Furthermore, they should also be free of substances toxic to humans and trace residual monomers. It is difficult for the polymers structure to meet these requirements simultaneously.1-3
The technology to produce SAHs with high water absorption and retention has been well developed. Researchers are also conducting studies on the absorption of blood. However, poly (sodium acrylate), a crucial example of a SAH in use, is not biodegradable. To solve this issue, many researchers are studying biodegradable SAHs, obtained using biomass materials such as starch, carboxymethyl cellulose, and sodium alginate.13-16
In recent years, bio-based polymer materials including those prepared using renewable resources have become more popular in order to solve environmental problems such as global warming and availability of materials. The research trends for substitution of acrylic or methacrylic acid in polymers main chain with bio-based counterparts is more active. Therefore, the market for bio-based products is growing rapidly and demand will increase. In addition, the exceptional properties of bio-based polymers are opening up new applications in polymer chemistry, pharmacy, and agriculture.
Itaconic acid (IA) is a compound rarely found in nature, but it can be produced industrially through the fermentation of carbohydrates such as lactic acid and starch, or the combustion of natural citric acid. Therefore, IA is listed as one of the “top value-added chemicals from biomass” by the US Department of Energy.17-19 Unlike acrylic acid and methacrylic acid, IA has different pKa (3.84 and 5.55) values.20 Because of the different pH values, it is possible to effectively control the pH of the medium to pass on the other adsorbates. Also, due to the presence of two carboxylic groups, IA can be used to produce a copolymer with an effective acidity to improve the adhesion properties and increase the latex stability of the final material. IA and its derivatives can undergo efficient radical copolymerization reactions because of their constituent vinyl groups. An overview of the properties of the IA and the response of possible structural changes has been provided by Tate (1970). IA can be regarded as α-substituted acrylic or methacrylic acid (see Fig. 1) and is an isomer of citraconic and mesaconic acid. It is stable at acidic, neutral, and weak basic conditions at standard temperatures. Isomerization of IA is not difficult, either.17-18,21
Eco-friendly research has been conducted by manufacturing polymers from natural resources to reduce the use of fossil resources and manufacturing biodegradable polymers. Researches on the biodegradable polymers through copolymerization using IA has been investigated. In 1999, the bio-degradability of crosslinked poly(IA-co-epoxybutane) was measured by a soil burial degradation field test over 120 days. In 2005, IA-based crosslinked prepolymer was synthesized and their biodegradability was determined by the measurement of the biochemical oxygen demand for 50 days.
In this work, we prepared a potentially biodegradable SAH using IA as the main monomer, and determined the suitable type and content of a crosslinker for the IA-based SAH. In addition, major factors affecting the absorption performance of SAH were investigated.
Materials and Methods
Itaconic acid (IA, Aldrich ≥ 99%), vinyl sulfonic acid (VSA, Aldrich 25 wt% in water), sodium hydroxide (NaOH, Samchun pure chemical 99%), and Kaolin (Aldrich) were used without further purification. Tetra(ethylene glycol) diacrylate (TTEGDA, Aldrich) and trimethylolpropane triacrylate (TMPTA, Aldrich) were used as crosslinkers after being passed through an alumina column to remove the inhibitor. Ethanol (Samchun pure chemical, 99.5%) was used as the quenching solution. High-purity nitrogen gas (Daesung Gas Co.) was used after being passed through a molecular sieve (4 Å)/manganese (II) oxide column. Ammonium persulfate (APS, Aldrich reagent grade 98%) and potassium persulfate (KPS, Aldrich reagent grade 98%) were used after recrystallization in ethanol.
IA and VSA were introduced into a 500 mL four-necked flask with a mechanical stirrer, a reflux condenser, a needle to introduce nitrogen gas, and a thermometer inserted through the injection ports. The mixture of monomers was partially neutralized with a sodium hydroxide solution in 30 mL of distilled water. The reactor was heated to 60°C in a nitrogen atmosphere. Then, the crosslinker and kaolin were carefully injected into the monomer mixture. A given amount of kaolin was slowly added to the monomer mixture under stirring. No precipitation or agglomeration of kaolin particles was observed. Keeping the temperature of the reactor constant, the initiator in a solution of distilled water (10 mL) was introduced into the reactor. The reaction was terminated when stirring became impossible due to the high viscosity of the hydrogel formed. The product was poured into ethanol for 12 h, and dried in a vacuum oven at 60°C for 12 h. Finally, we ground the sample and classified the particle sizes in the range of 300-500 μm. The crushed sample was dried in a vacuum oven at 60°C for 12 h. Figure 2 shows a summary of the experimental process to prepare the SAH composite.
Free absorbency (FA) is a fundamental measure for the absorption performance of SAPs. 200 mL of distilled water was added to the beaker, and NaCl was added to form a 0.9 wt% aqueous solution. 0.1 g of SAH particles accurately weighed using the parchment paper and carefully introduced into a 100-mesh wire netting tea bag. The tea bag was taken out of the 0.9 wt% NaCl solution after 30 min, and the water was wiped off the tea bag, which was then weighed. This method was measured according to EDANA, WSP 240.2.R3 (12). The FA was calculated using the following equation:
where W1 = weight of the swollen SAH particle and W0 = weight of the dried SAH particle.
The centrifuge retention capacity (CRC) is a measure of how much moisture is retained on dehydrating swollen SAHs with a centrifuge. Centrifugation of 0.1 g SAH sample was undertaken for 3 min at 300 G to dehydrate it. The centrifuged SAH was then weighed to evaluate the capacity of remaining water. This method was measured according to EDANA, WSP 241.2.R3 (12). The CRC was determined using the following equation:
where W1 = weight of the swollen SAH particle and W0 = weight of the dried SAH particle.
The dehydration ratio is an index indicating the amount of water removed on centrifugation: it indirectly predicts the gel strength. The dehydration ratio was calculated using the following equation:
Measuring the absorbency under load (AUL) is a method of determining the absorption capacity of SAH in the presence of a constant pressure. The AUL was determined as follows. A ceramic filter plate (d=80 mm, h=5 mm) was placed on a petri dish; then, a filter paper slightly larger than the plate was cut and placed on the ceramic filter plate. The tea bag was placed on the filter paper and the glass cylinder (d=60 mm, h=50 mm) in the center of the filter paper. 0.4 g of the dried SAH was sprayed on the inside of the cylinder evenly, and then the cylinder was pressurized with a piston (0.3 psi, h=50 mm). Taking care not to touch the ceramic filter plate, a 0.9 wt% NaCl aqueous solution was poured directly into the petri dish. The swollen SAH particles were weighed after 60 min. This method was measured according to EDANA, WSP 242.2.R3 (12). The AUL was calculated using the following equation:
where W1 = weight of the swollen SAH particle and W0 = weight of the dried SAH particle.
200 g of a 0.9 wt% NaCl aqueous solution was poured into a beaker. Accurately weighed 1 g of the SAH was placed in a beaker. The SAH particles were sufficiently swollen at 500 rpm for 16 h. Then, the fully swollen gel was allowed to deposit at the bottom. The supernatant was separated from the swollen gel with a filter paper. The supernatant was quantified and the theoretical amount of NaCl contained therein was calculated (WNaCl) assuming that WNaCl for a supernatant sample weighing 100 g is 0.9 g. After removing the water by distillation under reduced pressure from the supernatant, the weight (W) of the residue was measured. This method was measured according to EDANA, WSP 270.2.R3 (12) I then obtained the sol fraction using the following equation:
Figure 3 shows the ATR-FTIR spectrum for the prepared SAH. The broad absorption band at 3200-3600 cm−1 can be attributed to the hydroxyl groups in IA. The band observed at 1714 cm−1 can be attributed to C=O stretching owing to the carboxylate functional groups in the IA and TTEGDA. In the spectrum acquired for poly(IA-co-VSA), absorption peaks at 1182 and 1047 cm−1 appeared due to the sulfonyl group. The band at 1550 cm−1 was attributed to asymmetric C=O stretching, reconfirmed by another peak at 1449 cm−1, which was related to the symmetric stretching mode of the carboxylate group.22
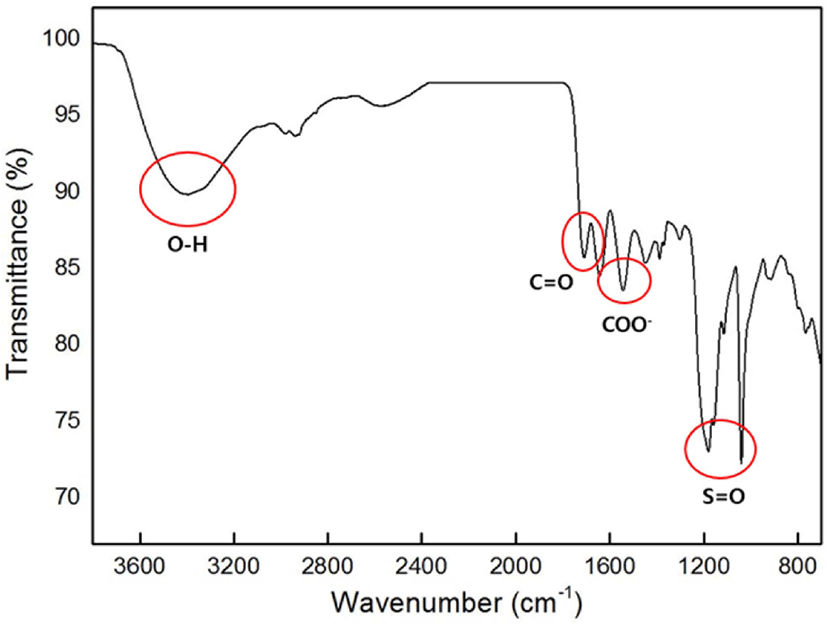
We investigated the performances of the SAH composites by varying different synthetic conditions such as the monomer molar ratio, polymerization temperature, degree of neutralization, the type and content of the initiator, and the type and content of the crosslinker. The CRC and AUL of the SAH for the various polymerization conditions were compared. In addition, variation in the sol fraction according to the polymerization temperature was determined. The dehydration ratio was also investigated to examine the effect of the crosslinker on the SAH.
Figure 4 shows changes in the CRC of the SAHs with variation in the molar ratio of IA with VSA. Compared with increase IA molar content, increase in the VSA molar content could show a better increase in the CRC of the SAHs. The AUL decreased over a reduction of IA 0.7 molar content (VSA 0.3 molar content or more). VSA contributed to the degree of polymerization of the copolymer and the increase of the sulfonyl group of VSA which has better neutralization efficiency than the carboxyl group.20,34 The initial sharp increase in absorption capacity due to neutralization efficiency is attributed to an increase in the internal osmotic pressure of the polymer and an increase in the anionic repulsion of the neutralized functional group. In addition, the sol fraction of the SAH was expected to decrease according to VSA molar content increase. However, the conversion of IA to VSA is low at increasing rates of CRC performance after IA 0.7 molar content due to reduced equivalence of ionic functional groups and consequent decrease in chain expansion. In addition, as the gel strength decreased due to the increase of VSA, the AUL decreased at VSA exceeding 0.3 molar content (less than IA 0.7 molar content). Therefore, all further studies were carried out at a molar ratio of IA:VSA=7:3, where the highest AUL values were observed. This is because a higher AUL can be obtained through additional processing steps (e.g., surface-crosslinking). Furthermore, it was selected according to the market demand that favored SAH with high AUL.
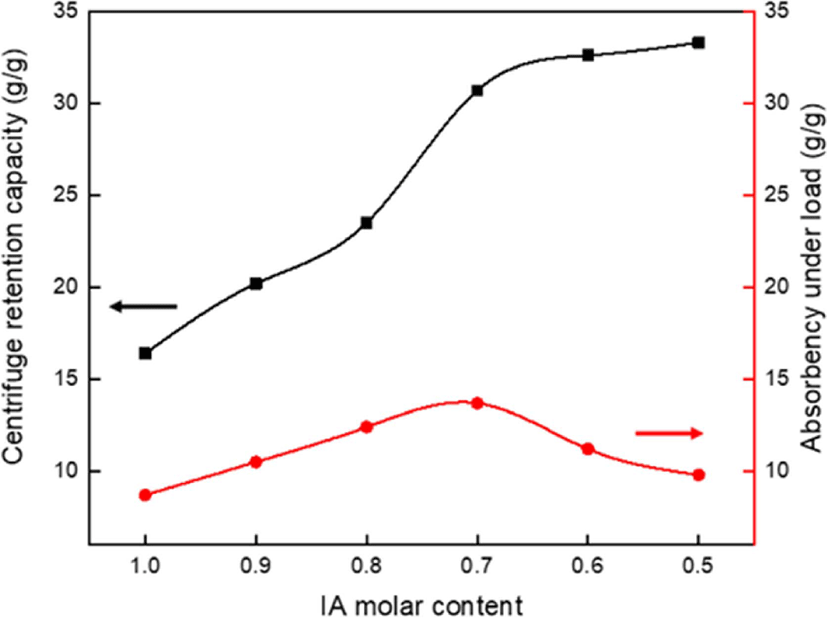
The effects of the polymerization temperature on the CRC and AUL of the SAH are shown in Figure 5. The CRC of the prepared SAHs increased as the polymerization temperature increased below the critical temperature. We reasonably inferred that the SAHs have the highest degree of polymerization at 60°C, which results attributable to the formation of a compact structure for absorption by enhancement in the crosslinking efficiency. The swelling ratio of crosslinked network increased with improve in the crosslinking efficiency according to Flory’s network theory.23-25
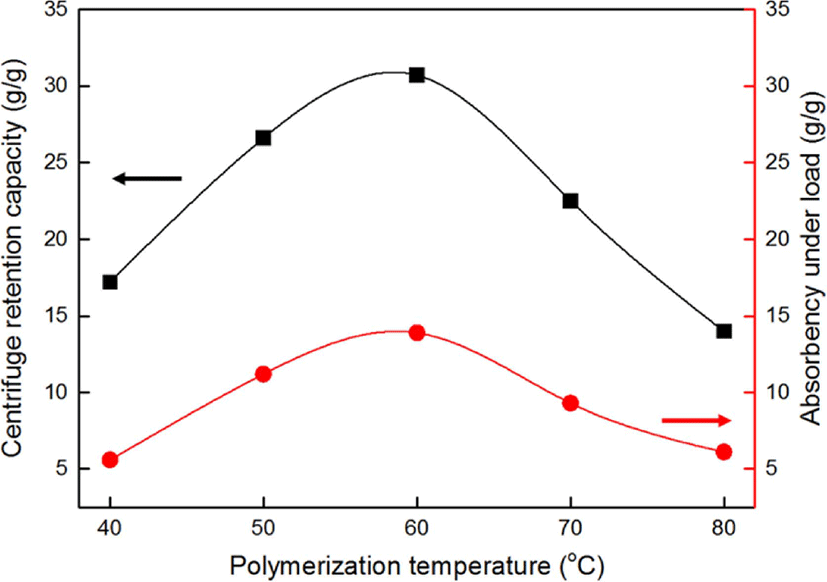
However, the CRC of the SAH decreased dramatically above the critical temperature of 60°C. When the reaction temperature increased above 60°C, the polymerization reaction rate increased and the degree of polymerization decreased. As the polymerization temperature increased, the number of radicals formed increased sharply, resulting in a decrease in the degree of polymerization, which denotes an increase in the number of chain ends, i.e., an increase in the number of low-molecular-weight linear chains that are not crosslinked.24-25
To verify this observation, we determined that variation in the sol fraction according to the polymerization temperature. Generally, low-molecular-weight polymer chains and non-crosslinking linear polymers that are not included in the polymer network are said to be soluble or extractable. As shown in Table 1, as the polymerization temperature increased, the sol fraction decreased below the critical temperature of 60°C. This means that the polymerization degree increased with increasing polymerization temperature.
Temperature (°C) | Sol fraction (%) |
---|---|
40 | 38.1 |
50 | 23.3 |
60 | 17.2 |
As for the AUL, the SAHs showed very similar trends as those noted for the CRC since the SAH surface characteristics were essentially the similar in this case, as well.
Figure 6 shows the effects of the neutralization degree of the monomers on the CRC and AUL of the SAHs. The CRC of the SAHs increased from 30 to 40 mol%, the critical neutralization degree, and decreased as the neutralization degree of monomers increased after 40 mol%. According to Flory’s network theory, the swelling ability of an ionic polymer network can be attributed to the ionic osmotic pressure and hydrophilic polymer chain. In addition, the carboxylate anions present in the polymer chain led to electrostatic repulsion, which can expand the SAH network. As a result, as the degree of neutralization below the critical point of 40 mol% increased, the CRC and AUL of the SAHs also increased.23
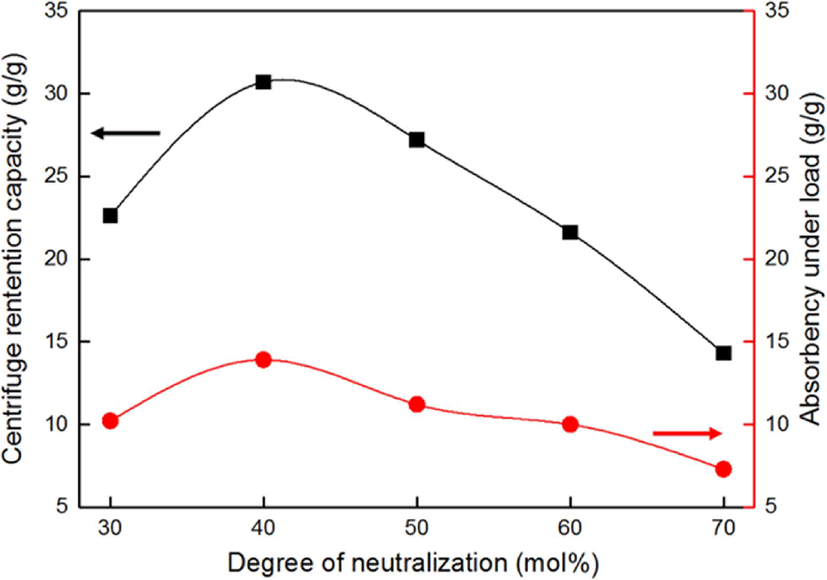
On the other hand, as the degree of neutralization increased further, the CRC of the SAHs decreased owing to the generation of excess Na cations, which reduced the carboxylate anions and prevented effective anion–anion electrostatic repulsion: thus, formation of the gel network was hindered, which resulted in the decrease in the CRC and AUL of the SAHs.
Figure 7 shows the effects of the type and content of the initiator on the CRC and AUL of the SAHs. The CRC and AUL of the SAHs increased for an increase in the initiator content from 0.25 to 0.75 mol/L and decreased with further increase in its value.
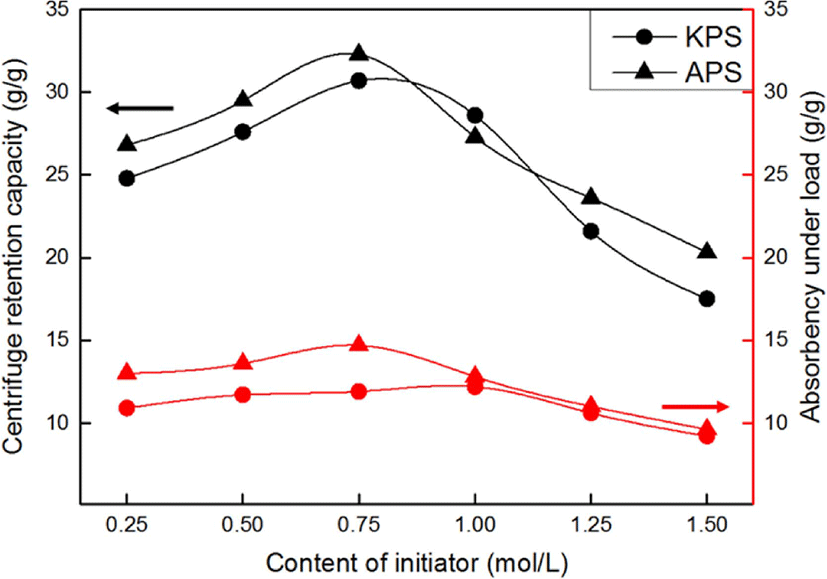
At the initiator content of 0.75 mol/L, the system was affected by the initiator content as a result of changes in the polymerization degree of the SAH. The degree of polymerization of the SAHs increased with increasing initiator content, resulting in enhancement of the crosslinking efficiency.
However, above the critical point of initiator content of 0.75 mol/L, The CRC and AUL of the SAHs decreased according to the theory elucidated below. The relationship between the average kinetic chain length (υ) and the concentration of the initiator in free-radical polymerization is given by the following equation:23
where Kp, ki and kt are the rate constants for propagation, initiation, and termination, respectively; f is the efficiency of initiation by the initiator; and [I] and [M] are the initial concentrations of the initiator and the monomer, respectively. According to Eq. (6), the molecular weight in free radical polymerization will decrease with an increase in the initiator concentration23-29
As the content of the radicals generated in the initiator increased, the termination reaction occurred frequently. With decrease in the molecular weight, the relative number of linear polymer chain ends increased. The linear polymer chain ends did not contribute to the water absorbency. Therefore, as the initiator content increased beyond the critical point, the CRC and AUL of the SAHs decreased.
Comparison of the results obtained using APS and KPS showed that the SAH produced using APS had a higher absorption capacity than the one fabricated using KPS. There are two reasons behind this. First, the radical half-life of APS is shorter than that of KPS at the same temperature. Furthermore, the affinities of the two species with water are different. As APS is more hydrophilic than KPS, it is more suitable for aqueous solution polymerization than KPS. In other words, APS offers a good initiating efficiency at the same radical concentration and temperature in aqueous solution polymerization.30
The crosslinker plays a major role in modifying the absorption performances of SAHs. I used two crosslinkers with different numbers of functional groups for our investigation, as shown in Table 2: TTEGDA is difunctional as it has two vinyl groups at the ends, while TMPTA is trifunctional owing to the three vinyl groups at the ends.
Difunctional crosslinker | Trifunctional crosslinker |
---|---|
![]() |
![]() |
Tetra(ethylene glycol) diacrylate (TTEGDA | Trimethylolpropane triacrylate (TMPTA) |
Figure 8 shows the effects of the type and content of the crosslinker on the CRC and AUL of the SAHs. The CRC of the SAHs decreased dramatically as the content of the crosslinker increased, irrespective of the crosslinker type. A higher crosslinker content resulted in a higher crosslinking density of the SAHs, leading to a decrease in the internal free space of the polymer network to retain the water. Our experimental data are consistent with Flory’s network theory.23-27
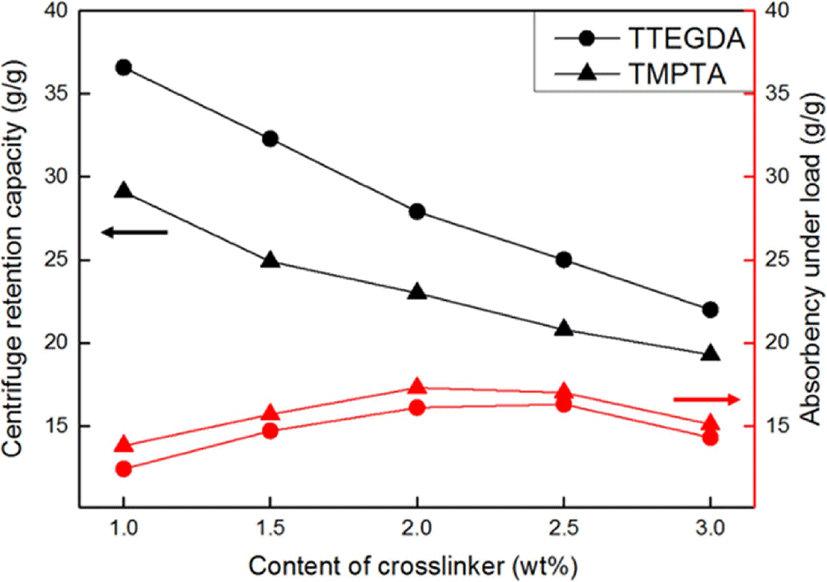
On comparing the results obtained using TTEGDA and TMPTA, the performance of the SAHs using TTEGDA was found superior than that achieved using TMPTA. Because TMPTA has three vinyl groups, the crosslinking density of the SAHs using TMPTA increased, leading to a decrease in the space left for water to enter. In other words, CRC values of the SAHs using TTEGDA were relatively higher than those of the SAHs using TMPTA, owing to the low cross-linking density of the latter.31-32
Other polymerization parameters such as the polymerization temperature, degree of neutralization, and type and content of the initiator showed similar trends in terms of the CRC and AUL of the SAHs, whereas the crosslinker content showed a different trend. In other words, as the crosslinker content increased, the CRC decreased and the AUL increased. This can be explained in terms of the gel strength. When the crosslinker content increased, the crosslinking density of the SAHs also increased, resulting in enhancement of the gel strength of the SAHs. Since the AUL of the SAHs is largely influenced by the gel strength, SAHs having a higher crosslinking density show a higher gel strength.33
However, a crosslinking density that is too high leads to a decrease in the AUL of the SAHs because of the lowered water absorption, as demonstrated by the CRC values of the SAHs. Therefore, it is important to control the optimal crosslinking density using the optimal crosslinker content to obtain superior performance for the SAHs.
Figure 9 shows the effect of the type and content of the crosslinker on the dehydration ratio of the SAHs. As the crosslinker content increased, the dehydration ratio of the SAHs decreased. Increasing the crosslinker content increased the crosslinking density of the SAHs. In addition, the SAHs prepared with TMPTA, having a high crosslinking density, showed a relatively low dehydration ratio.
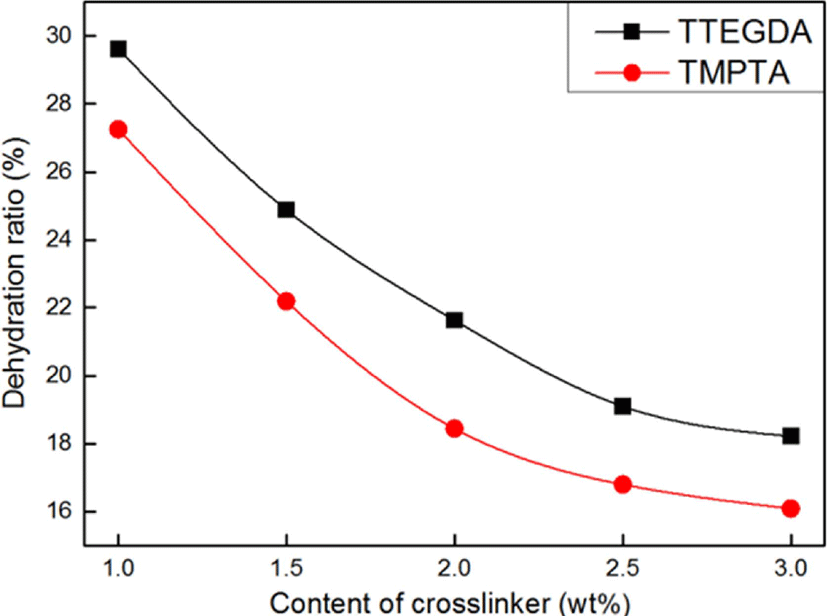
This tendency could be also explained by the difference in the crosslinking density of the SAHs depending on the type of crosslinker used. SAHs with a high crosslinking density can be said to improve the gel strength. Therefore, the dehydration content of TMPTA having trifunctional groups was lower than that of TTEGDA having difunctional groups, and the higher the amount of crosslinker used was, the higher was the gel strength.34
Conclusions
We prepared a SAH from the monomers itaconic acid (IA) and vinyl sulfonic acid (VSA), used in a molar ratio of 7:3, by aqueous solution polymerization using a thermal initiator. The structure of the SAH was characterized by ATR-FTIR. We also investigated the effects of the polymerization temperature, degree of neutralization, type and content of initiator, and type and content of crosslinker on the absorption properties of the SAH.
The main factors affecting the polymerization degree were the polymerization temperature and the initiator. The degree of neutralization contributed to the expansion of the SAH network structure by creating anions in monomers.
The content and type of crosslinker had an impact on the crosslinking density of the SAH. The crosslinker did not have a critical concentration regarding the CRC of the SAH. These results are different from those obtained for other polymerization parameters. However, in the case of the AUL of the SAH, it is important to select the optimum crosslinker content to control the crosslinking density of the SAHs.