Introduction
Polyurethane elastomers (PUEs) exhibit versatile mechanical properties as one can see many applications like coating, adhesive, sealant, sealant, and so on.1 The PUEs are basically composed of alternating hard and soft segments. Soft and hard segments are generally composed of polyether, polyester or polycarbonate-based glycol and diisocyanate and low molecular weight diol, respectively. The resulting polymeric PUE molecules form a microphase-separated structure depending on the thermodynamic immiscibility of the constituent segments. The hard segment chains occasionally are in the crystalline state with the formation of hydrogen-bonded. Since the molecular aggregation structure including the microdomain structure, the degree of microphase separation, and the hydrogen bonding state of hard segments are closely related to the mechanical property, investigation and control of the structure are quite important.
Since there are a very large number of starting materials for polymerization of PUE, the various properties can be tuned by choice of materials, conditions, molar ratio and so on. For example, poly(oxytetramethylene) glycol (PTMG), 4,4’-diphenylmethane diisocyanate (MDI) and 1,4-butanediol (BD)-based PUE is well-known as to exhibit superior mechanical property.2,3 To complement lack property, for instance, flexibility, low Young’s modulus, durability for light of PTMG-MDI-BD-based PUE, amorphous polyol, like poly(oxypropylene) glycol (PPG), cycloaliphatic/aliphatic diisocyanate4-6 and so on, should be employed. Recently, it was reported that trans-1,4-bis(isocyanatomethyl) cyclohexane (1,4-H6XDI) exhibits superior property in terms of mechanical property and durability for light.7-9 However, the structure-property relationship of the 1,4-H6XDI-based PUEs are not well understood and control of mechanical properties in the wide range has not been attained.
In this study, four types of 1,4-H6XDI-based PUEs were synthesized with various hard segment content and BD/TMP ratio. The relationship between structure and properties of the 1,4-H6XDI-based PUEs were studied by swelling test, infrared spectroscopy (IR), differential scanning calorimetry (DSC), wide-angle X-ray diffraction and small-angle X-ray scattering (WAXD and SAXS), dynamic viscoelastic property measurement and tensile testing.
Experimental section
Poly(oxytetramethylene) glycol (PTMG: number-average molecular weight (Mn)=1826, Asahi Kasei Chemicals Co., Ltd., Japan), trans-1,4-bis (isocyanatomethyl) cyclohexane (FORTIMO™ 1,4-H6XDI, Mitsui Chemicals Inc., Japan), 1,4-butanediol and 1,1,1-trimethylol propane (BD and TMP, Wako Chemicals Co., Ltd., Japan), were used as the polymer glycol, diisocyanate, chain extender, and cross-linking agent for polyurethane synthesis, respectively. BD was refluxed in the presence of calcium hydride to remove water and then purified by distillation. The amount of residual water was a few hundred ppm. FORTIMO™ 1,4-H6XDI was used without further purification. Impurity is chroline derivative in ppm.
The PUEs prepared in this study were synthesized in bulk with PTMG, 1,4-H6XDI and BD and TMP. Figure 1 shows the synthetic scheme of a prepolymer method. The 1,4-H6XDI-based PUEs with two hard segment contents, 20 and 30 wt%, and two TMP contents, 0 and 20 wt%, were synthesized. The nomenclature denotes the type of diisocyanate, hard segment content, and BD/TMP ratio, for example, HX-30-8/2 denotes 1,4-H6XDI-based PUE with hard segment content of 30 wt%, and BD/TMP = 8/2.
PTMG was dried with dry nitrogen under reduced pressure using a separable flask prior polymerization. Prepolymers were synthesized from PTMG and excess 1,4-H6XDI with a mechanical agitator and a formulation ratio of K=[NCO]HX/[OH]PTMG=3.0 and 2.0 for HX-30 and HX-20, respectively, at 80°C for 3 hours under a nitrogen atmosphere, where [NCO]HX and [OH]PTMG are the number of moles of NCO groups and OH groups, respectively. 10 mg of dibutyltin dilaurate (DBTL, Wako Chemical Co., Ltd. Japan) was added as a catalyst in the prepolymer reaction. The extent of the polymerization reaction was pursed by an amine equivalent method and the end of the reaction was determined by confirming that reaction ratio of NCO groups exceeded 90%. After completing the prepolymer reaction, the prepolymer was degassed using a vacuum agitator. BD or a mixture of BD/TMP were added to the prepolymer obtained and agitated in vacuo at a ratio of [NCO]pre/[OH]BD/TMP=1.03, where [NCO]pre and [OH]BD/TMP are the numbers of moles of NCO groups in the prepolymer and OH groups of BD and TMP, respectively. After mixing the viscous product for 90 s, it was poured into a mold constructed using a 1 mm thick spacer and two aluminum plates heated at 120°C. The PUEs were cured at 120°C for 24 h in air.
The swelling behavior of the 1,4-H6XDI PUEs was evaluated with toluene and N,N-dimethylacetamide (DMAc). Toluene and DMAc are non-polar and polar solvents, respectively. Prior to soak the sample to solvents, specimens were dried in vacuo for 24 hours. To swell the PUEs, specimens were soaked in each solvent at 60°C to attain the equilibrium swelled state. The gel fraction, G, was defined as G=Wb/W, where Wb and W are the dry weight after swelling and initial weight, respectively. Moreover, the degree of swelling, Q, of the PUEs was determined from weights of dry and swollen PUE samples. Q is defined as following equation, Q=1+ [(Wa-Wb)/ds/(Wb/dp)], where Wa, ds and dp are the weight of sample swollen to equilibrium state, the density of solvent, and density of the PUEs, respectively.
The state of hydrogen bond of the hard and soft segments in the 1,4-H6XDI-based PUEs was evaluated by attenuated total reflection Fourier transform infrared spectroscopy (ATR-FT-IR). Spectra were obtained with an FTS-3000 EXCALIBUR (Bruker Japan Co., Ltd. Japan) equipped with a liquid N2 cooled mercury cadmium telluride (MCT) detector using a ATR cell (MIRacle, PIKE Technologies, Inc. USA). All spectra were collected with 32 scans and at a resolution of 4 cm−1.
DSC measurement was performed to investigate glass transition temperature and melting point of the PUEs. The DSC curves were obtained using a DSC (Rigaku DSC 8230, Rigaku Denki Co. Ltd. Japan) in the temperature from −140 to 250°C under a nitrogen atmosphere. A heating rate was 10°C min−1. At the start of measurement, as-prepared samples were simply cooled down to around −145°C.
Small-angle X-ray scattering (SAXS) and wide-angle X-ray diffraction (WAXD) measurements were conducted for the PUEs at the BL03XU8,9 and BL05XU beamline in the SPring-8 facility in Japan. The photon flux was ~1013 photons/ s, and the size of beam at the sample was 150 mm × 150 mm. The wavelength of X-ray was 0.1 nm. For SAXS measurements, a CCD detector (ORCA-R2, Hamamatsu Photonics, K. K.) with a pixel size of 126 mm/pixel and a total size of 672 × 512 pixels was used to measure the scattered radiation. The detector was placed behind a vacuum path, and the camera length was ca. 2 m. For WAXD measurements, a flat panel detector was employed, and the camera length was 63 mm. Scattering patterns were collected for 200~500 ms. Data obtained were reduced from the 2D pattern to 1D profile with FIT2D (Ver. 12.077, Andy Hammersley). Both sets of data were expressed using the wave vector, q, where q = 4π Sin θ/λ. Absolute scattering intensity was obtained by calibration with pure water.10
The temperature dependence of dynamic viscoelastic properties were obtained with a DMS 6100 (Seiko Instruments, Co., Ltd., Japan) in the temperature range from –150 to 250°C with a heating rate of 2°C min−1 under a nitrogen atmosphere. The sample size was 25 × 5 × 2 mm3. Imposed strain and frequency were 0.2% and 10 Hz, respectively.
Results and Discussion
Appearances of all PUEs are milky opaque. This implies that crystallites are formed from the hard segment chains in the all PUEs. Table 1 shows density, gel fraction and degree of swelling of the PUEs. Gel fraction of the PUEs swollen by toluene was greater than 99%. This indicates that a well-developed network structure was formed in all PUEs. Also, gel fractions of HX-20-8/2 and HX-30-8/2 with chemical cross-linking points by DMAc was greater than 98%. This implies that a chemical cross-linking network was properly formed by incorporation of TMP. Furthermore, HX-20-10/0 was soluble in polar solvent, DMAc, in contrast to HX-30-10/0. The linear PUEs, which form only physical cross-links, dissolve in DMAc, because hydrogen bonds in physical cross-links dissociate when solvated by molecules of polar solvent.4,11-14 Thus, insolubility of HX-30-10/0 in DMAc suggests that HX-30-10/0 forms quite strong physical cross-links. The degree of swelling in toluene and DMAc for PUEs decreased with increasing hard segment content. This simply corresponds to the increase of cross-linking region, in other words, increase in cross-linking density. The degree of swelling of HX-30-8/2 and HX-20-8/2 by toluene exhibited greater value than for HX-30-10/0 and HX-20-10/0. For the MDI-based PUE, incorporation of TMP induces decrease in the degree of swelling due to additional incorporation of chemical network structure.12 The reason why the 1,4-H6XDI-based PUE showed opposite trend to MDI-based PUE is associated with the strong cohesive force of hard segment chains, -(1,4-H6XDI-BD)n-. That is, incorporation of TMP induced great decrease of ordering hard segment chains for the 1,4-H6XDI-based PUE.
ATR-FT-IR measurements were carried out in order to evaluate hydrogen bonding state of the urethane carbonyl groups of hard segment chains and ether oxygen of soft segment chains in the PUEs. Figure 2 shows ATR-FT-IR spectra of (a) 3600~3200 cm−1 and (b) 1800~1600 cm−1 region for the four 1,4-H6XDI-based PUEs prepared with various hard segment ratio and BD/TMP ratio. NH and carbonyl stretching bands (n(NH) and ν(C=O)) were clearly observed at around 3300 and 1700 cm−1, respectively, for all PUEs. In all spectra, NCO stretching band was not observed at 2260 cm−1, which indicates that the curing and cross-linking were properly occurred for all PUEs. The carbonyl stretching bands at 1660 cm−1 which is characteristic of allophanate groups were not also observed. The hydrogen bonding state in the hard segment chains can be ascertained from the absorbance of the ν(NH) and ν(C=O) bands.15,16 NH stretching bands forming hydrogen bonds with ether oxygen and carbonyl groups and free one are generally observed at around 3290-3310, 3300-3350 and 3450 cm−1, respectively. On the contrary, the hydrogen bonded carbonyl stretching band (ν(C=OH-bond)) and free one (ν(C=Ofree)) are observed at around 1690 and 1725 cm−1, respectively. Thus, bands observed at 3340, 1693 and 1721 cm−1 in Figure 2 are likely to correspond to ν(NH), ν(C=OH-bond) and ν(C=Ofree) bands, respectively. The ratios of Iν(C=OH-bond) to Iν(C=Ofree) (Iν(C=OH-bond)/Iν(C=Ofree)) for HX-30-10/0 and HX-20-10/0 were almost the same and much higher than for HX-30-8/2 and HX-20-8/2. Furthermore, the ν(NH) band for HX-30-10/0 and HX-20-10/0 observed at 3340 cm−1 were sharper than for HX-30-8/2 and HX-20-8/2 and shifted to higher wavenumber region. These results clearly indicate that the hard segment chains of HX-30-10/0 and HX-20-10/0 are well-organized and more strongly hydrogen bonded in comparison with HX-30-8/2 and HX-20-8/2.
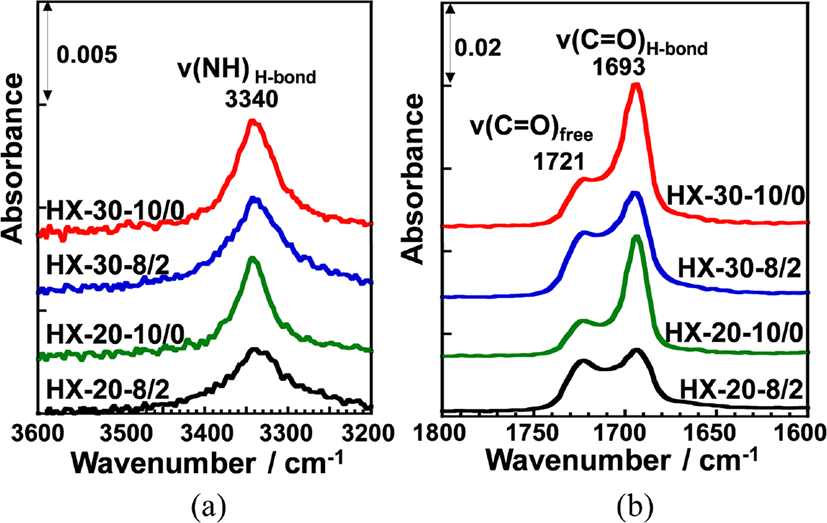
Thermal behaviors of the PUEs were investigated by DSC. Figure 3 shows the DSC thermograms for the 1,4-H6XDI-based PUEs prepared with various hard segment contents and BD/TMP ratio. Baseline shift and endothermic peaks were observed at around −70, 0, and 180°C for all PUEs. These can be assigned to the glass transition temperatures of the soft segment chains (Tg,S), melting of crystallized soft segment chains (Tm,S), and melting points of the crystallized hard segment domains (Tm,H s). The Tg,S and Tm,S decreased with increases in hard segment content and decrease in TMP content. It is well known that the increasing Tg of the soft segment in PUE systems means an increasing miscibility of the two components on account of the molecular interaction between the soft and hard segments. Therefore, it is likely to consider that the soft segment phase with the richest state is in HX-30-10/0. On the contrary, for the hard segment chains, Tm,H increased with increase in hard segment content and TMP content. Increase in TMP content seems to give appropriate length of hard segment chains to crystallize. Thus, it seems reasonable to consider that the degree of microphase separation became stronger with increase in hard segment content and decrease in BD content.
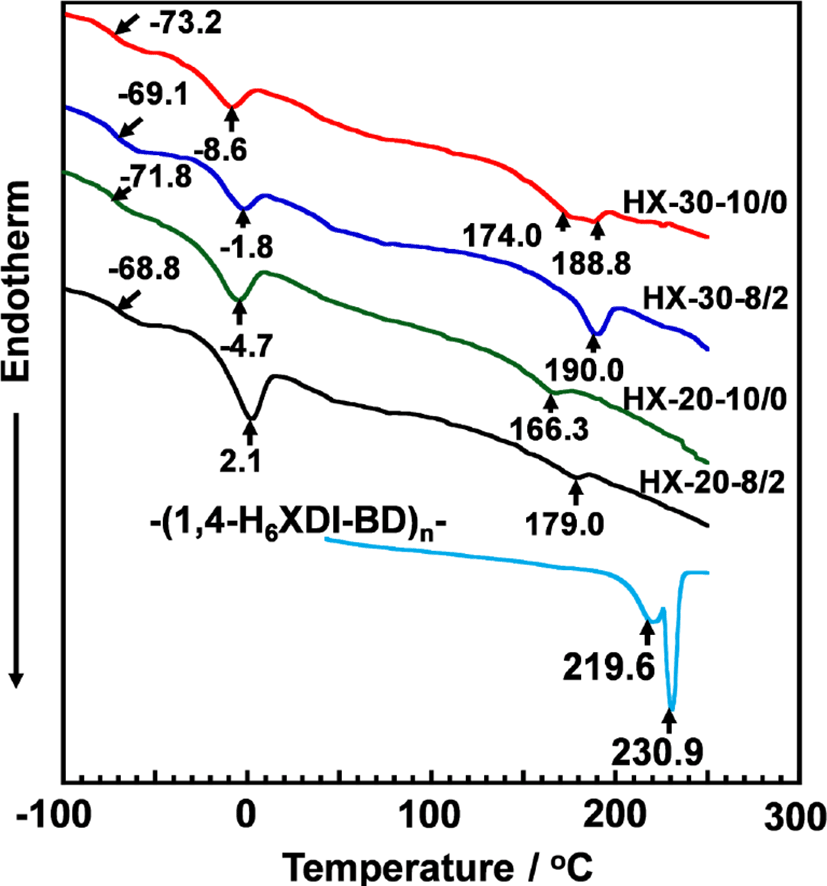
To investigate the crystal structure of the hard segment chains in the PUEs, WAXD measurements were carried out. Figure 4 shows WAXD profiles for the 1,4-H6XDI-based PUEs prepared with various hard segment contents and BD/TMP ratios. For comparison, the WAXD profiles of hard segment models for -(1,4-H6XDI-BD)n- were also plotted. The crystalline peaks were clearly observed at q = 12.0, 13.9, 15.2 and 17.0 nm−1 for the -(1,4-H6XDI-BD)n- model. These peak positions correspond well to those obtained for triclinic of P1 symmetry with a = 0.736 nm, b = 0.861 nm c = 1.784 nm, α = 140.9°, β = 142.56°, γ = 33.9°.7 For HX-30-10/0, HX-30-8/2 and HX-20-10/0, one can clearly see crystalline peaks at the same positions with the hard segment model, though some of them are difficult to recognize because of overlapping amorphous halo of soft segment. It is conceivable that the hard segment chains are packed in the same crystal lattice even in the PUEs. The intensity of crystalline peak of HX-30-10/0 was much higher than that for HX-20-10/0. This is due to the formation of well-organized crystallized hard segment domains as well as increase in hard segment content.
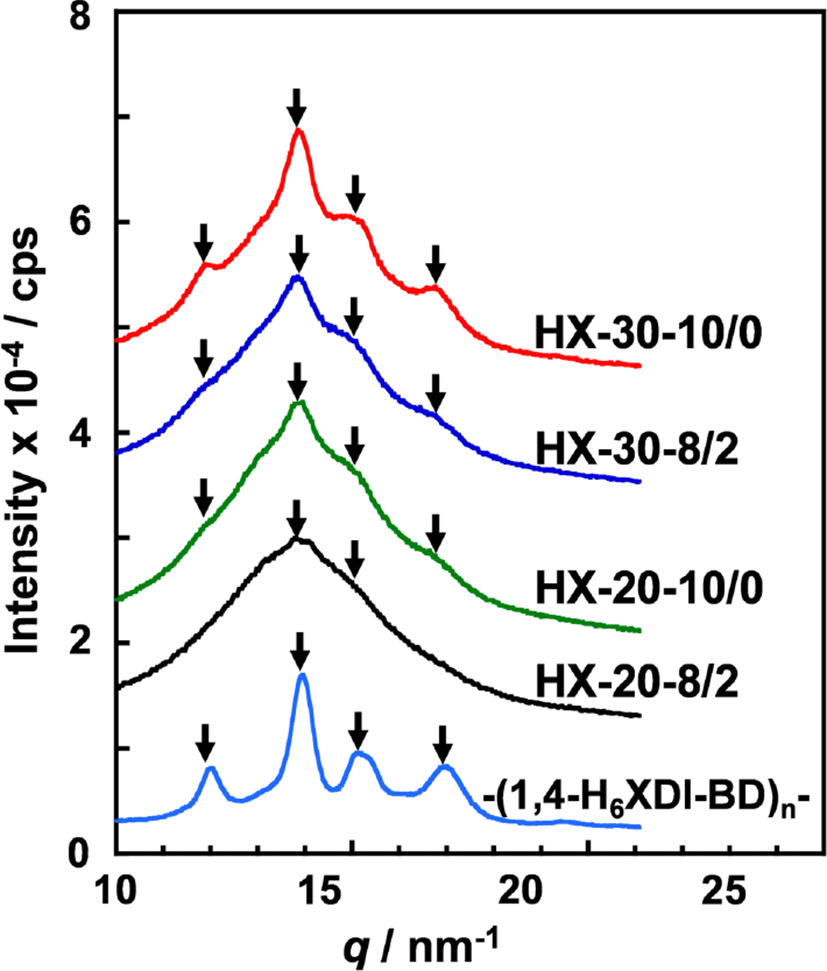
The degree and size of microphase separated structure in the PUEs was investigated by SAXS measurement. Figure 5 shows 1D-SAXS profiles for the 1,4-H6XDI-based PUEs prepared with various hard segment contents and BD/TMP ratios. Broad scattering peaks observed in the q range from 0.1-0.8 nm−1 are associated with interdomain spacing of hard segment domains in a microphase-separated structure, which is comprised of the isolated hard segment domains and a surrounding soft segment matrix. Peak positions of HX-30 PUEs were lower than for HX20. To discuss in detail, interdomain spacings were determined by analyzing the three-dimensional correlation function. Moreover, diffuse phase boundary thickness of hard and soft segments was determined by analyzing the deviation from Porod’s law. These quantities were obtained according to the calculation methods by Bonart et al.17 and Koberstein et al.18Table 2 summarizes interdomain spacings calculated from peak positions using Bragg’s law and determined by correlation function analysis. The relative degrees of overall microphase separation for the HD-30 were larger than for HD-20.
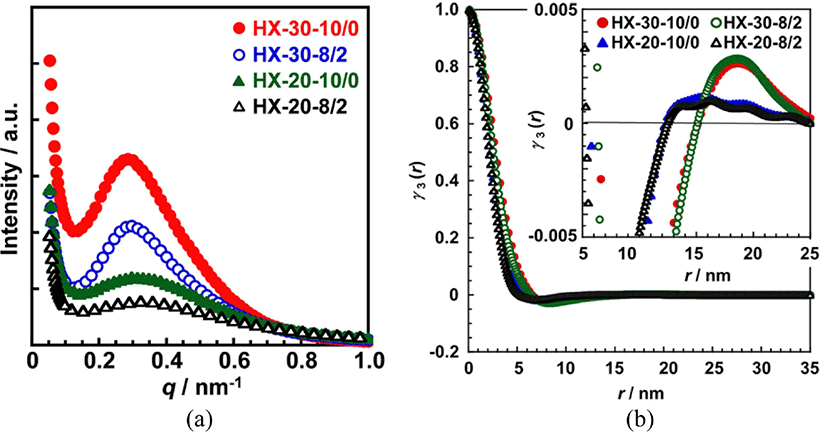
The boundary diffuseness (Δη2”/Δη2’-1), sigmoidal and linear boundary thicknesses (σ and E) of HX-30 were slightly larger than for HX-20. Moreover, the magnitudes of HX-30-8/2 were smaller than for HX-30-10/0 and HX-20 did not show clear trend. The PUEs containing large amount of hard segments form larger hard segment domains, and they show larger boundary diffuseness. Incorporation of TMP reduced the size of hard segment domains, resulting decrease in boundary diffuseness and thickness. The boundary diffuseness and thickness are affected by the length and its distribution of hard segments, an aggregation force, and formation ability of hard segment domains.
Figure 6 shows dependence of the dynamic storage modulus (E’), loss modulus (E”), and loss tangent (tanδ) of the 1,4-H6XDI-based PUEs on temperature with various hard segment contents and BD/TMP ratios. The E’ and tanδ values of all 1,4-H6XDI-based PUEs steeply decreased and increased at around −70°C during the heating process. These changes are associated with the glass transition of the soft segments. Above the glass transition of the soft segment chain, a shoulder in E’ curve at around −40°C was observed. This can be assigned to recrystallization of the soft segment. On the contrary, two dispersions were clearly observed from 100 to 0°C in the tanδ curve. They correspond to the glass-rubber transition and recrystallization of the soft segment chains during the heating process as discussed above. The rubbery plateau region was observed from 0 to 150°C for all the PUEs. The order of the E’ value at the rubbery plateau region was as follow: HX-30-10/0 > HX-30-8/2 > HX-20-10/0 > HX-20-8/2. Further discussion on the E’ values at rubbery plateau region will be given with Young’s modulus by stress-strain curve. The terminal temperatures of HX-30 were higher than for HX-20, and also the temperatures of HX-30-8/2 and HX-20-8/2 were higher than for HX-30-10/0 and HX-20-10/0. In other words, increase in hard segment content and incorporation of TMP increased terminal temperature. These results are quite consistent with the fact that increase in hard segment content and TMP content increased Tm,H as observed by DSC.
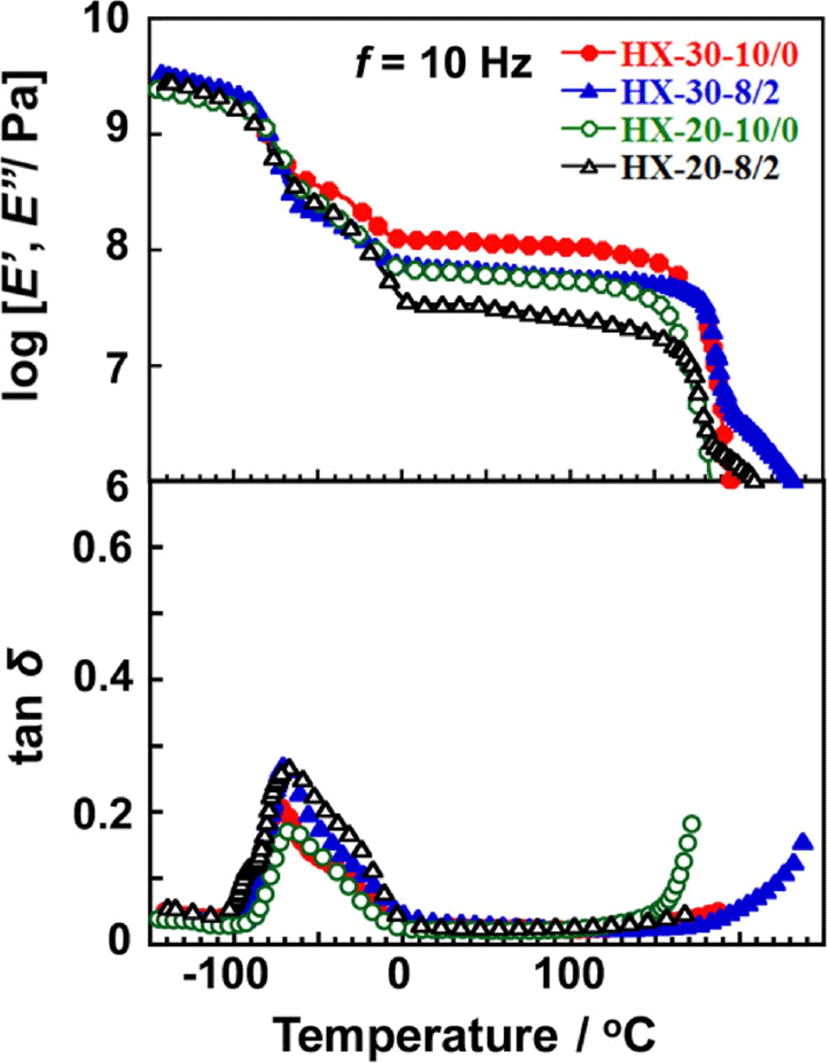
Figure 7 shows stress-strain curves of the 1,4-H6XDI-based PUEs with various hard segment contents and BD/TMP ratios. The Young’s moduli estimated from an initial slope of stress-strain curves, tensile strength and strain at break value for the PUEs are summarized in Table 3. Young’s modulus and tensile strength increased with an increase in hard segment content. Young’s modulus decreased with increasing TMP content. HX-30-8/2 and HX-20-8/2 exhibited much larger slope in the larger strain region in comparison with HX-30-10/0 and HX-20-10/0. This is due to decreasing size of well-developed hard segment domains induced and chemical cross-linking points existing in the soft segment matrix by incorporation of TMP. For HX-30-10/0 and HX-20-10/0, the slope in the larger strain region were quite small and strain at break were around 10. HX-30-10/0 and HX-20-10/0 possess well-developed cylindrical hard segment domains.7 Thus, it is likely that obvious breaks of anisotropic cylindrical hard segment domains might have occurred for HX-based PUEs.
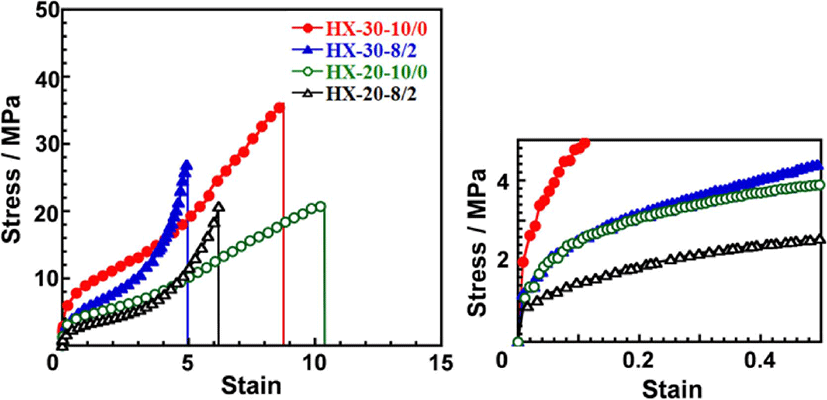
Sample | Young’s modulus/MPa | Strain at break | Tensile strength/MPa |
---|---|---|---|
HX-30-10/0 | 19.1 | 8.8 | 36.6 |
HX-30-8/2 | 11.4 | 5.0 | 27.4 |
HX-20-10/0 | 8.0 | 14.5 | 18.5 |
MD-20-8/2 | 6.1 | 6.1 | 20.7 |
Conclusions
Four 1,4-H6XDI-based PUEs with two different hard segment contents (20 and 30 wt%) and BD/TMP ratios (10/0 and 8/2) (HX-30-10/0, HX-30-8/2, HX-20-10/0, and HX-20-8/2) were synthesized with PTMG as a soft segment. The hard segment chains in the 1,4-H6XDI-based PUEs crystallized easily forming strong intermolecular hydrogen bonds due to the symmetry of 1,4-H6XDI molecule. It was revealed that the degree of microphase-separation increased with an increase in hard segment content and BD ratio. Young’s modulus and strain at break were controlled in the ranges between 6 to 20 MPa and 5 to 15, respectively. These values are comparable with those for commercial 4,4’-diphenylmethane diisocyanate (MDI)-based PUEs, which are the one of the most popular PUEs in terms of mechanical properties. Furthermore, 1,4-H6XDI’s lack of aromatic moieties is expected to greatly enhance color stability of resulting PUEs.7,19 All the above features suggest 1,4-H6XDI could replace MDI in a range of applications.