Introduction
Superabsorbent polymers (SAPs) are hydrogels containing hydrophilic three-dimensional network structures that maximize their swelling properties to provide an absorption capacity of 10 to 1000 times by weight depending on the medium to be absorbed.1 The SAPs are widely used in the field of hygiene, e.g. as water-absorbing materials in disposable diapers and women's sanitary goods where they can capture secreted fluids such as urine, blood, etc.1 Meanwhile, a broad range of non-hygiene-related applications include their use as water-swellable rubbers, drug-delivery systems,2 soil additives for agriculture, environmental sensors, etc.1,3,4
Recently, various studies have been aimed at achieving enhanced permeability and absorbability with smaller material loadings of SAP. Although the gel strength can be improved by the application of techniques to increase the internal crosslinking density and surface crosslinking, the absorption capacity is concurrently decreased because the network width becomes narrower as the gel strength increases. Therefore, it is important to select the appropriate monomer and find the optimal polymerization conditions for achieving the maximum performance in this trade-off relationship. In general, SAP with high performance in various absorption characteristics is prepared by the fast polymerization of acrylic acid (AA). However, AA-based SAP has the disadvantages of skin toxicity and lacks environmental friendliness.3,4 Consequently, many researchers have studied the synthesis of SAP using biomass-derived materials in place of AA.5-7
In the present study, SAP is prepared via the terpolymerization of itaconic acid (IA), vinyl sulfonic acid (VSA), and cellulose in order to limit the use of environmentally harmful materials. In particular, this route replaces the conventional petrochemical-derived AA with IA, a biomass-derived material having two carboxyl groups and one vinyl group that enables radical polymerization.8-10 The IA is produced by fermentation of carbohydrates such as lactic acid and starch. In addition, the absorption characteristics of the SAP is supplemented by the addition of the strongly polar hydrophilic VSA as a comonomer.11,12 The resulting increase in the number of anionic groups can increase the resistance of SAP towards salinity and increase its absorption capacity.13,14 The second comonomer, cellulose, is a natural polysaccharide formed by plant photosynthesis,15 thus providing biological properties such as biocompatibility and nontoxicity.16,17 Cellulose can be modified by oxidation to partially substitute the hydroxyl groups with carboxyl groups. The presence of the carboxyl groups provides the oxidized cellulose with good hy-drophilicity along with the potential for transformation into macro alkoxy radicals in the presence of initiators in order to initiate chain propagation of the vinyl group. The addition of cellulose also improves the gel strength.18
After determining the optimal terpolymer composition and quantity of inner-crosslinker, the obtained core-SAP (CSAP) was then surface-crosslinked by esterification with butane diol for the optimal surface crosslinking time to improve its liquid permeability and absorbency under load (AUL).
Experimental
Itaconic acid (IA, Junsei, special grade) and vinyl sulfonic acid (VSA, Sigma Aldrich) were used as a monomer without purification. cellulose was purchased from the Daesang (South korea) and used without purification. Poly(ethyleneglycol) diacrylate (PEGDA, Sigma Aldrich), 1,6-hexanediol diacrylate (HDODA, Sigma Aldrich) were used as the inner-crosslinker, Sodium persulfate (NaPS, Sigma Aldrich) was used as the initiator, and 1,4-Butanediol (BD, Sigma Aldrich, 99%) was used as the surface-crosslinker without purification.
IA, VSA and cellulose according to the total content of 100 g were added to a four-neck reactor and 80 g of distilled water was added to maintain a nitrogen atmosphere. 50% NaOH aqueous solution corresponding to 70% of the total molar ratio of total monomers was slowly added to the reactor. After temperature is stabilized less than 50°C, 0.2 g of HDODA and PEGDA as inner-crosslinker were added and mixed uniformly. Thereafter, 0.5 g of NaPS was added to the reactor and stirred for 2 h. Then, the reaction was transferred to a plastic tray and dried in a vacuum oven at 60°C for 24 hours. The dried product was frozen in a 20°C freezer and then pulverized with a grinder. The crushed product was spread evenly on a wide tray and dried at 60°C for 12 h. The dried product was classified into a particle size of 300 to 600 μm. We showed synthetic composition of CSAP and abbreviation of SAP made of poly(itaconic acid-ter-vinyl sulfonic acid-ter-cellulose; PIVC).
Table 2 shows the composition of the surface-crosslinking solution and surface crosslinking time for the preparation of SSAP. Methanol, distilled water and BD were mixed in a ratio of 6.1:2.9:1 to prepare a surface-crosslinking solution in a 20 ml vial. 3 g of CSAP was added to the surface crosslinking solution and swelled. The swollen CSAP was transferred to an aluminum tray and reacted at 165°C for 15.0-25.0 minutes. After that, in order to remove unreacted monomers, the surface-crosslinking step was completed after washing with acetone and drying.
FT-IR (Nicolet, NEXUS) was used to analyze the structure of SAP. To examine the thermal stability of CSAP, thermo gravimetric analysis (T.A. Instruments TGA Q500) was performed. The specimens were conducted on TGA analyzer under N2 atmosphere with a heating rate of 20°C/min in the temperature range of 25-800°C. The sample weight loss was analyzed as a function of temperature.
CRC is a value indicating how much water is retained after dewatering the swollen SAP with a centrifuge. 0.1 g of SAP was swollen in 0.9 wt.% NaCl aqueous solution for 30 minutes, then dehydrated to 300 G in a centrifuge and weighed. CRC was obtained using Equation (1), where ω1 = the weight of the swollen SAP particles and ω0 = the weight of the dried SAP particles.
AUL is a value that indicates how much moisture the SAP can absorb under a given pressure. As in the measurement of free absorbance, 200 mL of distilled water was poured into a beaker, and NaCl was added thereto to prepare a 0.9 wt.% NaCl aqueous solution. A total of 0.16 g of the SAP was evenly distributed in the prepared cylinder, and the weight was taken using 0.3 psi weights. Then, a ceramic filter plate was placed on a chalet, and filter paper was placed on it. The cylinder containing SAP was placed on the filter paper, and sufficient aqueous solution of NaCl was added. After 1 hour, the cylinder was taken off, and the weight was measured with the weights. AUL was obtained using Equation (2), where ω1 = the weight of the swollen SAP particles and ω0 = the weight of the dried SAP particles.
Permeability is a measure of the amount of water flowing between swollen SAP layers. First, 0.5 g of SAP was added to the equipment and SAP was swollen for 30 minutes by adding a sufficient amount of 0.9 wt.% NaCl solution. A 0.3 psi pressure device was installed on the swollen SAP. The cock at the bottom of the device was opened and the time taken for the 20 mL 0.9 wt.% NaCl solution to pass was measured.
Results and Discussion
The CSAP was prepared by the radical polymerization of neutral monomers in the presence of an inner-crosslinker and radical initiator. The presence of the carboxyl group (−COOH) makes IA strongly hydrophilic, while radical polymerization can occur via the vinyl groups. In addition, VSA is a hydrophilic monomer with strong polarity. The initiator is decomposed into the persulfate radical anion, which abstracts a hydrogen atom from the cellulose hydroxyl group to form the more active alkoxy radical. This then reacts additively with the IA monomer to form the polymer chain via propagation, during which crosslinking occurs via reaction of the growing polymer chain with the inner-crosslinker. The chemical synthesis of PIVC CSAP is shown in Scheme 1. The content of IA was fixed at 80 wt.% and the sum of VSA and cellulose was adjusted to 20 wt.%. The two inner-crosslinkers (HDODA and PEGDA were used together in equal amounts ranging from 0.4 to 0.6 g, as shown in Table 1.
The surface of the PIVC CSAP was crosslinked using BD to improve the AUL and permeability. The two hydroxyl (−OH) groups in BD take part in an esterification reaction with the −COOH groups of IA and cellulose. This reaction occurs as desired on the surface of the PIVC SAP particles and has no significant effect on the inside of the SAP particles. As a result, it is possible to increase the gel strength and surface crosslinking density while maintaining the size of the internal PIVC CSAP network.
Figure 1 shows the Fourier transform infrared (FT-IR) spectra of IA, VSA, cellulose, and PIVC CSAP. The presence of IA is verified by the characteristic absorption peaks at 2800-3200 cm−1, which are associated with the −COOH groups in the IA monomer. The absorption peak at 1721 cm−1 is associated with the C−O and C=O stretching vibrations. The presence of the characteristic S=O absorption band at 1219 cm−1and the −SO2−O stretching vibration at 1184 cm−1 is confirmed for the VSA monomer. The IR spectrum of cellulose displays the O−H stretching absorption in the region of 3550–3200 cm−1, the C−H stretching at 2930 cm−1, and the glycosidic ring C–O–C and C−O−H stretching absorptions at 1015 cm−1. By comparison, the PIVC SAP displays absorption peaks at 3550–3200 cm−1 due to the O−H group and at 3000 cm−1 due to −COOH from IA and cellulose, along with each of the peaks identified above for the monomers, thus demonstrating the successful synthesis of the PIVC CSAP.
As indicated by the TGA curves in Figure 2, both the PIVC CSAP and PIV CSAP exhibit the first weight loss within the tem-perature range of 0-100°C due to loss of internal pore water followed by water that was chemically bound to the polymer chains.19 The two samples also show similar decomposition ratios of IA at around 400°C, while the decomposition temperature of the cellulose-containing terpolymer is about 4°C higher. The final weight loss step that occurs at 500-800°C may be due to the decomposition of the PIV CSAP and PIVC CSAP polymer backbone. Given that a moderately higher monomer crosslinking density is expected in the PIVC CSAP relative to that in the PIV CSAP, the results in Figure 2 demonstrate an increase in the thermal stability with increasing crosslinking density, in agreement with the results of Elif et al. and Ruma et al.20,21 In addition, the improved thermal stability of the PIVC CSAP indicates the successful incorporation of cellulose into the CSAP.22,23
The variations in the CRC and AUL with varying content of inner-crosslinker in the CSAP are indicated in Figure 3. Theoretically, the size of a polymer network is determined by the crosslinking density, which primarily depends on the content of crosslinker.24 Thus, the CRC is seen to decrease, and the AUL increase, as the inner-crosslinker content is increased from 0.4 to 0.6 g.
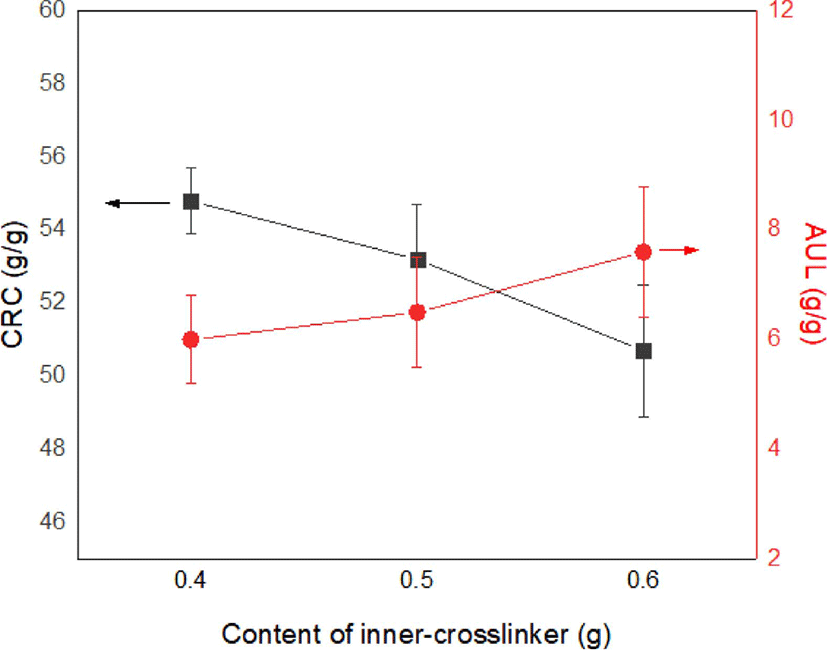
Due to surface crosslinking of the above-mentioned CSAPs under the conditions listed in Table 2, excellent CRC and AUL results are obtained with inner-crosslinker contents of 0.4 g or 0.5 g (Figure 4(a)). However, the liquid permeability of the SSAP at 120 s is not as good with 0.4 g of inner-crosslinker as compared to 0.5 g of inner-crosslinker; hence, the latter is regarded as the optimum condition for SSAP (Figure 4(b))
CSAP (g) | Surface-crosslinking solution composition | Temperature (°C) | Time (min) | ||
---|---|---|---|---|---|
Methanol (g) | DW (g) | Surface-crosslinker | |||
BD (g) | |||||
3.0 | 6.1 | 2.9 | 1.0 | 165 | 15.0 17.5 20.0 22.5 25.0 |
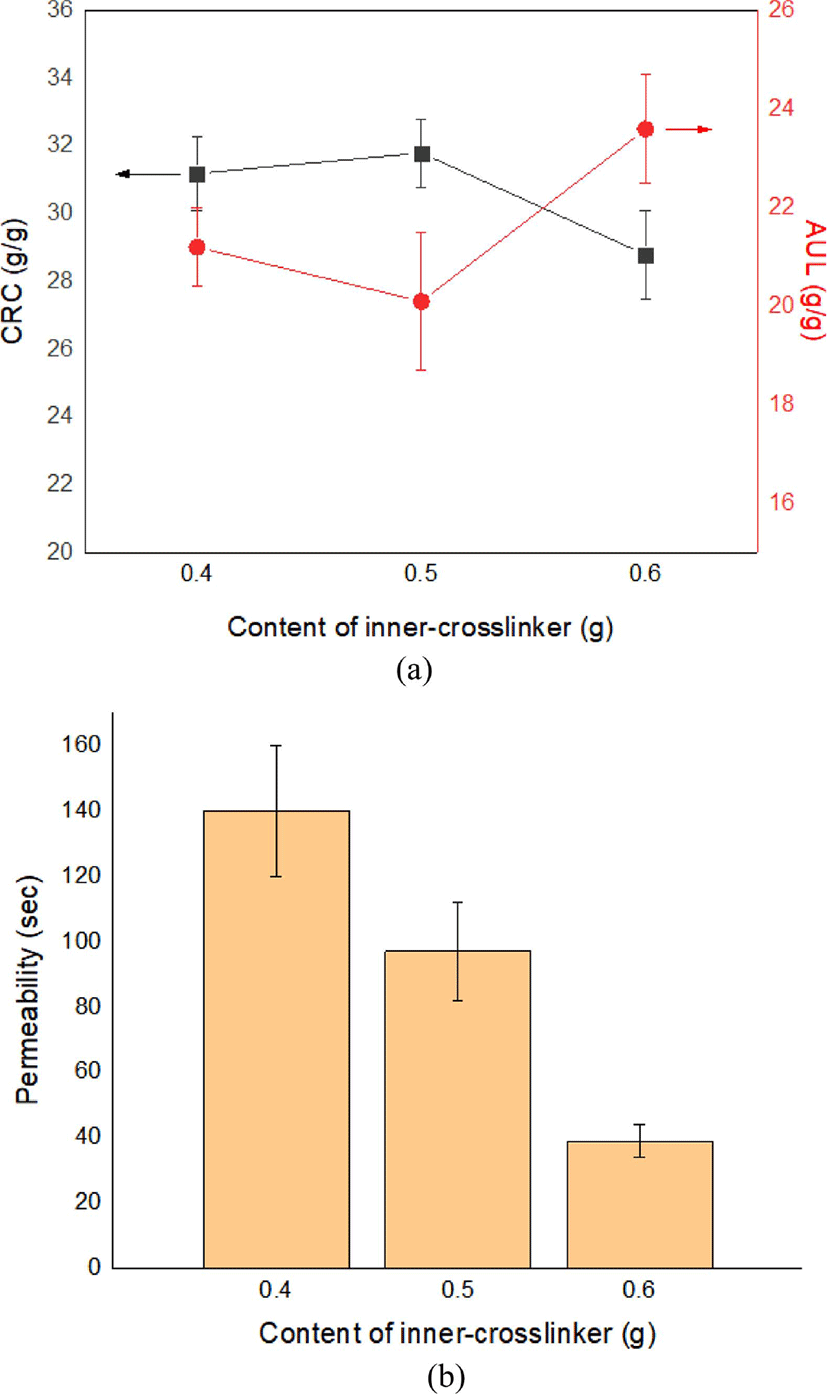
The effect of varying the PIVC cellulose content from 0 to 20% on the water absorbency of the PIVC SSAP is indicated in Figure 5. Here, the CRC is seen to initially increase with the addition of 5% cellulose and then gradually decrease with further increase in the cellulose content. Thus, a PIVC cellulose content of 5% is seen to be the optimum composition in terms of both CRC and AUL (~32 g/g and ~20 g/g, respectively; Figure 5(a)) and in terms of liquid permeability (~100 s; Figure 5(b)). Due to its small molecular weight, large specific surface area, high dispersibility in aqueous media, and multiple −OH groups, the uniform dispersion of cellulose in the SAP network increases the hydrophilicity and permeability of the porous SAP structure. A number of studies have been reported in SAP to improve various properties by applying biomaterials similar to cellulose.25-27
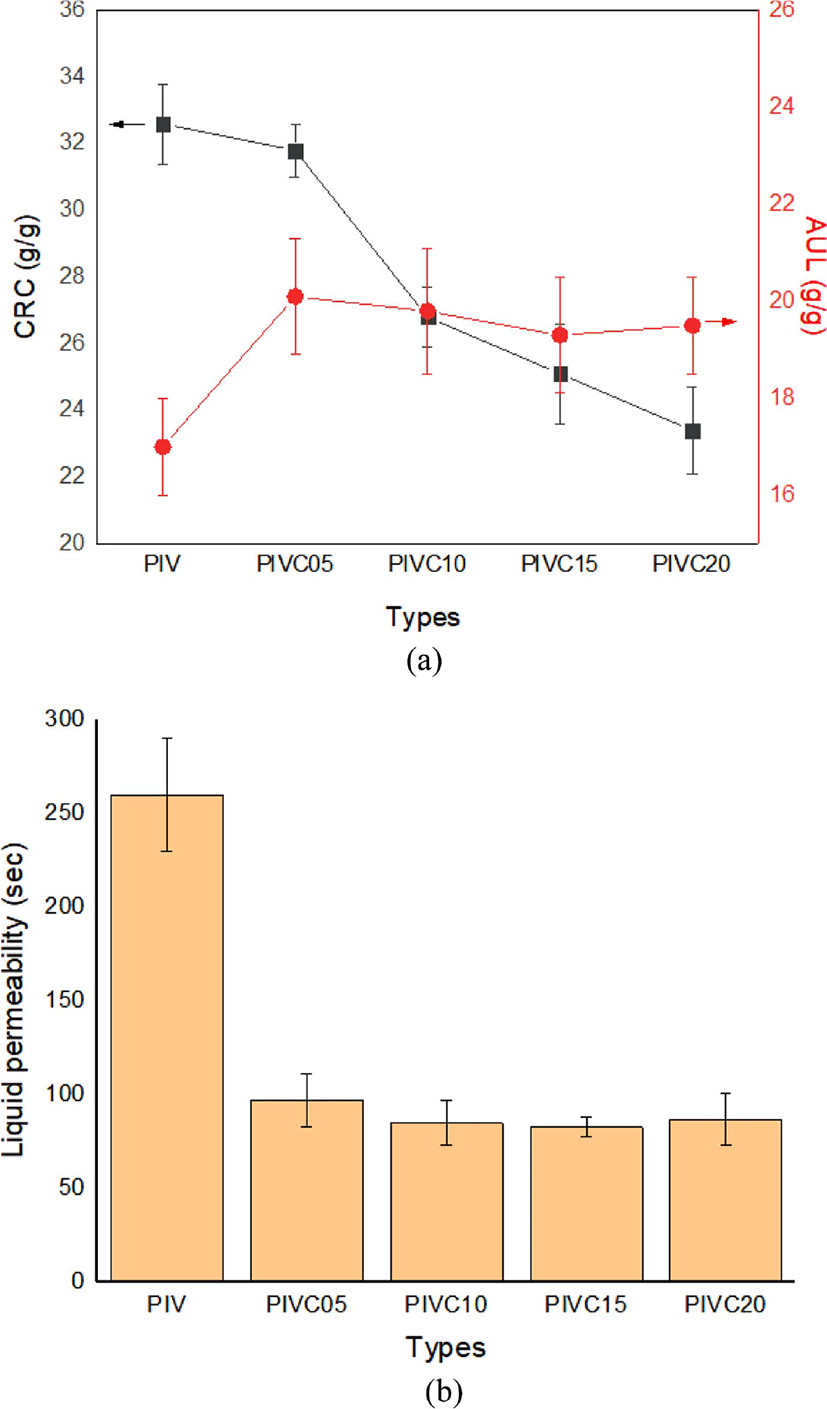
The esterification between CSAP’s carboxylic acid groups and the hydroxyl groups of surface crosslinking agent promotes an increase in crosslink density of the CSAP's surface which lowers the CRC. Crosslink density of the CSAP’s surface is related to various conditions such as types and contents of surface crosslinking agent, and composition of surface crosslinking solution, etc. As the surface crosslinking process is performed at high temperature, and the IA is vulnerable to excessive heat, the surface crosslinking time is another important factor that must be carefully selected. With this in mind, the absorption performance of the as-prepared CSAP and SSAP according to the surface crosslinking time is indicated in Figure 6. Here, it can be seen that a low surface crosslinking time (e.g. 15 min) leads to an incomplete SSAP network and no enhancement in the AUL and liquid permeability. When the network structure is narrowed by crosslinking processing, the gel strength of the SAP improves and affects AUL and liquid permeability.28 By contrast, a long surface crosslinking time leads to a narrower SSAP network and a decreased CRC. Thus, the results in Figure 6 indicate an optimal surface crosslinking time of 20 min. The optimal surface crosslinking time increases the probability of reaction between hydroxyl groups of 1,4-butanediol and carboxylic acid groups of CSAP leading to an increase in cross-link density on the SAP surface.
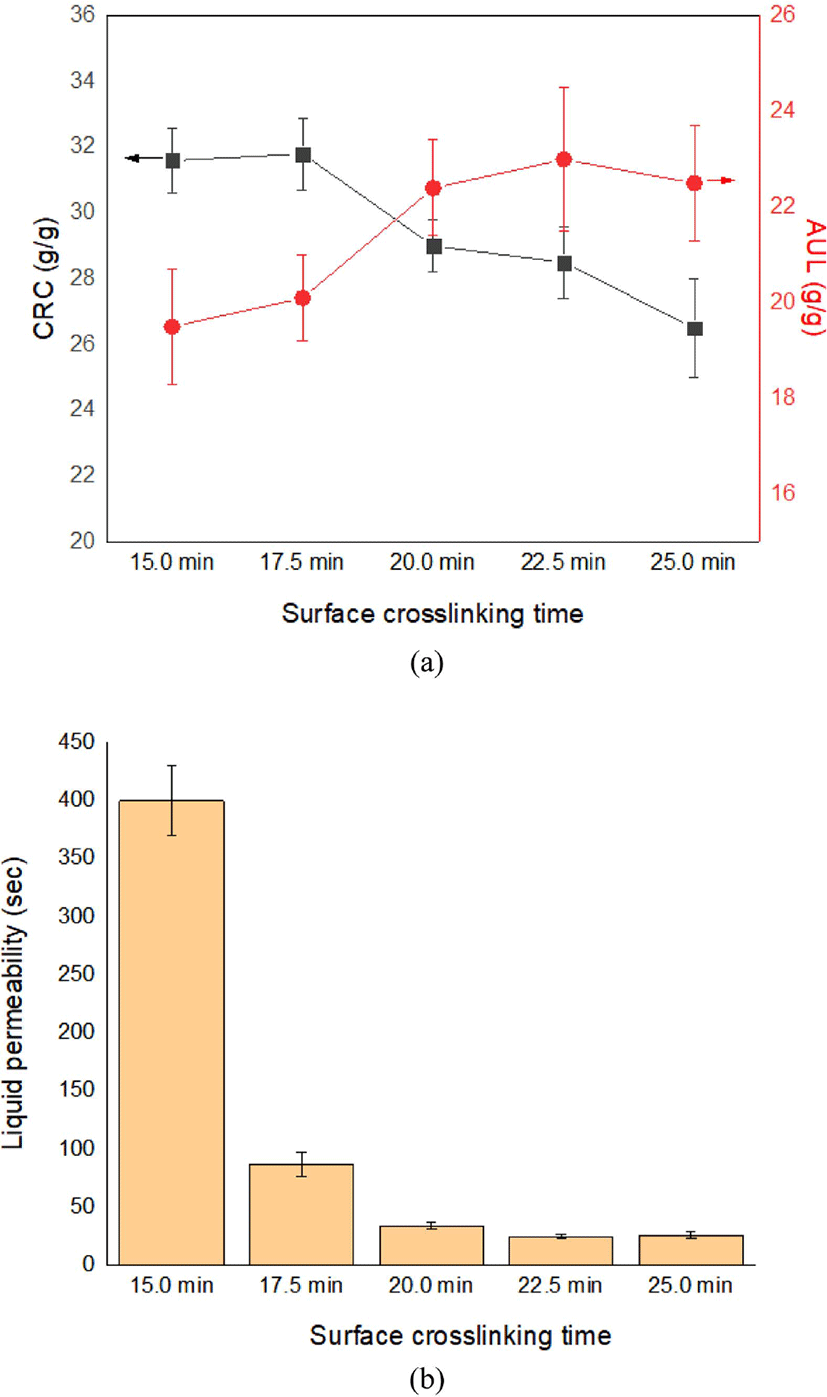
Conclusions
In this study, a range of superabsorbent polymers (SAPs) was prepared via terpolymerization of itaconic acid (IA), vinyl sulfonic acid (VSA) and cellulose. The absorption characteristics of these PIVC CSAPs were then compared according to the composition ratio of monomers. The amount of water retained after centrifugation, i.e. the centrifuge retention capacity (CRC), was found to increase as the VSA content was increased. This was attributed to the strong negative charge on the sulfonic group of the VSA. In addition, the absorbency under load (AUL) was found to decrease with increasing CRC, such that an inner-crosslinker content of 0.5 g was found to be the most suitable. Thus, after crosslinking, the PIVC050.5 SSAP sample showed the highest AUL and CRC values.
Further, the influence of the surface crosslinking time upon surface absorbency was determined by fixing the contents of inner-crosslinker and cellulose in the PIVC CSAP. Thus, a surface crosslinking time of 20 min led to enhanced AUL and permeability values of 22.5 g/g and 39 s, respectively, along with an excellent CRC of 28.5 g/g.
Overall, the optimal conditions for the SAP containing IA, VSA, and cellulose were shown to provide a similar level of per-formance to that of commercially available SAPs. Hence, the new PIVC CSAP is suitable for a range of practical SAP applications.