Introduction
In recent years, advanced oxidation processes (AOPs), including Fenton oxidation, photocatalysis, sonocatalysis, and ozonation, have been developed as promising alternative methods for the removal of hazardous pollutants from wastewater.1-5 Many studies have shown that sonocatalysis is the most attractive treatment for the degradation of organic pollutants compared with other AOPs, owing to its several advantages, such as eco-friendliness, low cost, convenient operation, and high efficiency.6-8 In the ultrasonic cavitation process, the microbubbles produced by ultrasonic waves provide a high temperature and high pressure environment for the pyrolysis of H2O molecules via continuous nucleation, growth, and collapse.9 As a result, ●OH radicals having strong oxidation ability are generated, which can subsequently break down a number of organic pollutants into smaller molecules and finally mineralize them into CO2 and H2O.10-12
MoS2 has a graphene-like two-dimensional structure which can offer a large specific surface area and excellent adsorption performance.13,14 Moreover, as a transition metal dichalcogenide (TMD), MoS2 has attracted tremendous attention and is widely applied in many fields, such as photoelectronics, hydrodeoxygenation (HDO), photocatalysis, and energy devices, owing to its chemical stability, good conductivity, high photosensitivity, and high carrier mobility.15-17 Over the past few decades, various techniques such as sonolysis, chemical vapor deposition, plasma microwave, and solvothermal or hydrothermal methods have been studied for the preparation of MoS2 nanoparticles.18-20 Notably, a decrease in nanocatalyst particle size indicates an increase in surface area, which causes shifts in the valence and conduction band levels.21 As a result, the indirect band gap energy is increased from a bulk MoS2 value of ~1.2 eV to a nanoscale MoS2 value of ~1.8 eV.22 In our previous work, we investigated the photocatalytic and catalytic behavior of MoS2 in aqueous media.23 Although many studies on the application of pure and modified MoS2 nanomaterials toward the degradation of organic pollutants have been conducted, to the best of our knowledge, the optimization of azo dye degradation via ultrasonic treatment has not been widely conducted.
Hence, this study aimed to investigate the morphology, microstructure, and elemental composition of as-synthesized MoS2 nanoparticles and to optimize various conditions, namely, sonocatalyst dosage, dye concentration, and ultrasound treatment time for the sonocatalytic degradation process of azo dyes, represented by Orange II. Response surface methodology (RSM) is a statistical method used to determine the optimal conditions in a multi-factor system, which has been widely used in the statistical analysis of wastewater treatment process conditions.24,25 In this study, RSM experimental design was employed with the Box–Behnken model to obtain the optimum conditions for sonocatalytic degradation of Orange II.
Experimental
Sodium molybdate dihydrate (Na2MoO4·2H2O) was obtained from Junsei Chemical Co. Ltd. Thioacetamide (TAA, CH3CSNH2) was purchased from Tokyo Chemical Industry Co., Ltd. 1-Methyl-2-pyrrolidone (NMP) was obtained from Samchun Chemicals. Hydrochloric acid, ethanol, and Orange II were purchased from Sigma-Aldrich, Inc. All chemical materials with commercial AR purity were used directly without further purification.
In a typical synthesis, 24 mmol of TAA and 4 mmol of sodium molybdate dihydrate were dissolved in an aqueous solution consisting of 100 mL deionized water and 15 mL ethanol. After vigorous stirring for 10 min at room temperature, the mixture was maintained at 90 °C for 5 min. The aqueous solution was then cooled to 80 °C, and 22.5 mL HCl (12 M) was added while stirring. After the addition of HCl, the color of the solution turned dark blue and then quickly turned dark brown. The reaction was maintained at 80 °C for 10 min, and the resultant precipitate was washed several times with deionized water to eliminate additional ions. After drying the precursor at 100 °C for 12 h, it was ground into a powder using a mortar and pestle. Finally, the powder was calcined in an electric furnace (Ajeon Heating Industry Co., Ltd.) at 850 °C for 2 h under an inert argon atmosphere to obtain the MoS2 nanoparticles.
Powder X-ray diffraction (XRD, Bruker, D8 ADVANCE) analysis with Cu Kα radiation (λ=1.54178 Å) was performed to identify the structural and crystalline phases of the MoS2 nanoparticles. The lattice vibrations of the products were characterized via Raman spectroscopy (BWS465 i-Raman Plus) using a laser operating at an excitation wavelength of 532 nm. The chemical states of the MoS2 nanoparticles were analyzed by X-ray photoelectron spectroscopy (XPS, ESCALAB 250 Xi, Thermo Fisher Scientific) using a monochromatized Al K-alpha X-ray source. The surface morphology was observed using scanning electron microscopy (SEM, Hitachi S-4800). Transmission electron microscopy (TEM) images was conducted using a JEOL JEM-2100 Plus instrument operating at 200 kV.
For sonocatalytic degradation experiments, ultrasonic treatment was conducted using a Cole-Parmer ultrasonic processor (20 kHz, 500 W). The temperature of the ultrasonic bath was maintained at 25±2 °C using a circulating water bath. For the sonocatalytic reaction, 5-15 mg/L of Orange II were prepared, and the performance of the as-synthesized MoS2 nanoparticles was evaluated by adding 0.2-0.6 g/L of sonocatalyst to 50 mL of the dye solution via ultrasonic treatment. To achieve an adsorption-desorption equilibrium between the sonocatalyst and Orange II, the mixture was stirred magnetically for 30 min in the dark condition before the ultrasonic treatment.
The Orange II degradation efficiency was determined as follows:
where C0 is the initial concentration of Orange II, and Ct is the concentration of dye after reaction time t.
To optimize the conditions for the sonocatalytic degradation of Orange II, Box–Behnken design (BBD) was applied with three factors at three levels using Design Expert 12 software. According to the BBD method, 17 runs were performed to evaluate the effects of sonocatalyst dosage, dye concentration, and ultrasound treatment time. The levels of different optional variables and RSM designs are listed in Table 1. Response variable (Y) is the degradation efficiency of Orange II and listed in Table 2. The correlation between the response variable and independent variables is expressed by a quadratic polynomial equation, written as follows26:
Independent variables | Units | Symbol | Range and Levels | ||
---|---|---|---|---|---|
-1 | 0 | 1 | |||
Sonocatalyst dosage | g/L | X1 | 0.2 | 0.4 | 0.6 |
Dye concentration | mg/L | X2 | 5 | 10 | 15 |
Ultrasound treatment time | min | X3 | 90 | 120 | 150 |
where β0, βi, βii, and βij are the constant coefficient, linear interaction coefficient, quadratic interaction coefficient, and cross-factor interaction coefficients, respectively, and X1, X2, and X3 represent the independent variables as the coded values of sonocatalyst dosage, dye concentration, and ultrasound treatment time, respectively.
Results and Discussion
XRD was performed to determine the crystalline structure and phase purity of the prepared MoS2 nanoparticles. As shown in Figure 1, peaks are observed in the XRD pattern at 14.33°, 32.76°, 39.64°, 49.70°, and 58.33° corresponding to the (002), (100), (103), (105), and (110) planes of the hexagonal phase of MoS2, respectively (JCPDS No. 37-1492).19 The absence of other peaks indicates that MoS2 was successfully synthesized with a high purity. The crystallite size of the MoS2 nanoparticles was calculated using the Scherrer equation:
where K is the shape factor considered as 0.9, λ is the wavelength of powder XRD with Cu Kα radiation (λ=1.54178 Å), β is the full width at half maximum (FWHM, β=0.51˚), and 2θ is the angle between the incident and scattered X-rays. Using this formula, the crystallite size of as-synthesized MoS2 nanoparticles at (002) plane was determined to be 15.71 nm.
Figure 2 shows the Raman spectra of the MoS2 nanoparticles. Two distinct Raman peaks are observed at 376 and 402 cm-1 corresponding to the in-plane E12g and out-of-plane A1g vibrational modes, respectively.27 The presence of the E12g and A1g vibrational modes implies that the samples have a 2H-MoS2 structure. In addition, a 36 cm−1 discrepancy in the Raman shift between the E12g and A1g vibrational modes indicated that as-synthesized MoS2 had good crystallinity and was multilayered.28
UV-vis absorption spectroscopy was performed to investigate the optical properties of the as-synthesized MoS2 nanoparticles. To obtain a well-dispersed solution, MoS2 powder was dispersed in the organic solvent of N-methylpyrrolidone (NMP) via ultrasonic treatment for 2 h. The UV-vis spectra of the MoS2 nanoparticles are shown in Figure 3(a). The absorption peaks were observed at 469, 624, and 675 nm. The peak at 469 nm is attributed to a threshold transition between the valence and conduction bands.29 The band-gap energy of the MoS2 nanoparticles was determined using the Tauc equation30:
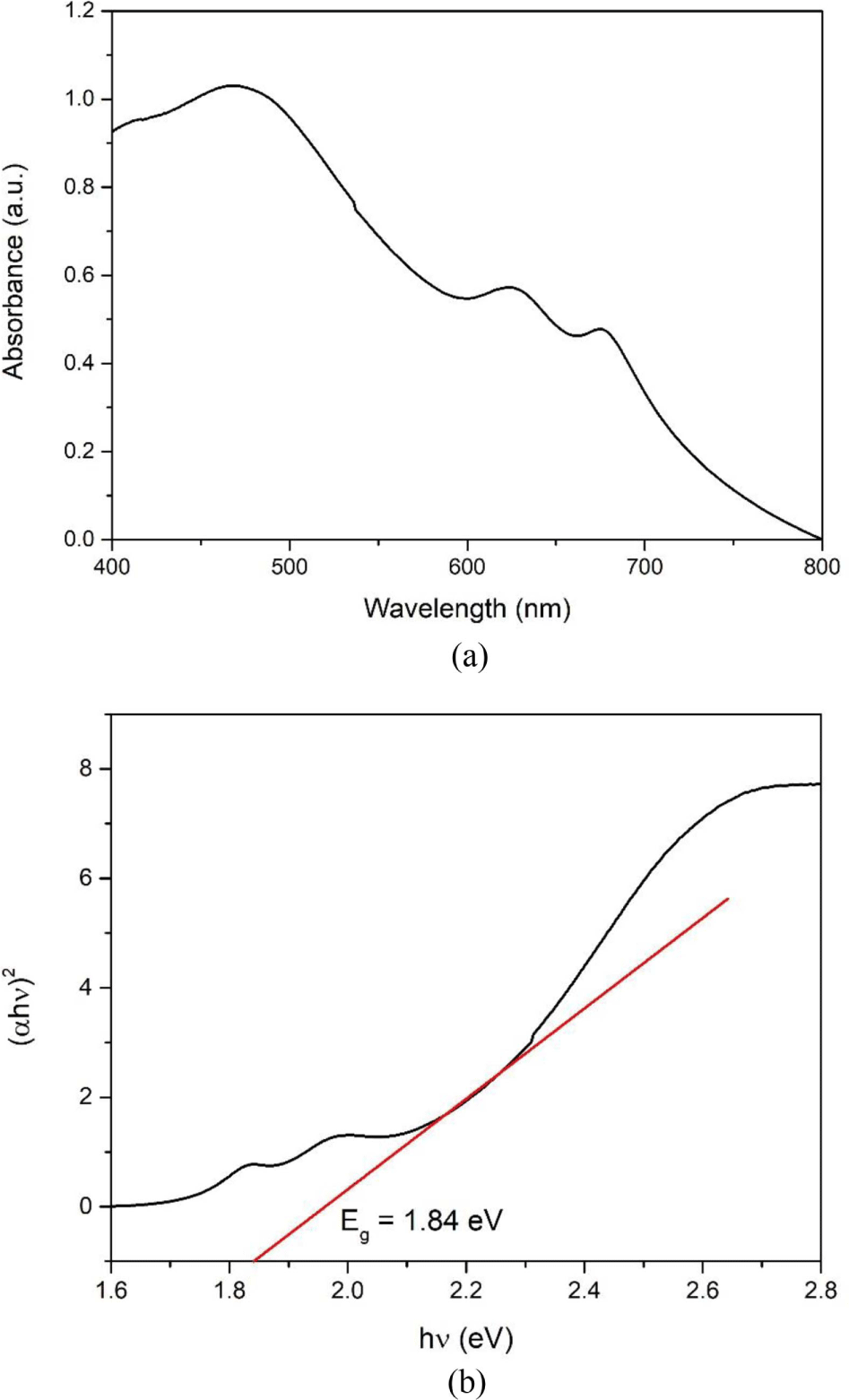
where α, h, and ν are the absorption coefficient, Plank’s constant, and the light frequency, respectively. B is a constant and Eg is the band-gap energy. As shown in Figure 3(b), the band-gap energy of the as-synthesized MoS2 nanoparticles was 1.84 eV.
The electronic states of the samples were identified using XPS analysis. The survey spectrum of the MoS2 nanoparticles in Figure 4(a) shows three major peaks corresponding to Mo 3d, S 2p, and C 1s. The C 1s peak observed at 284.81 eV can be attributed to the hydrocarbons in the XPS system and a small amount of carbon residue on the surface of the samples generated during the synthesis process.31 The Mo 3d spectrum in Figure 4(b) shows two strong peaks with binding energy values of 229.83 and 233.03 eV corresponding to Mo 3d5/2 and Mo 3d3/2, respectively, revealing the presence of Mo4+ in the samples.32 In Figure 4(c), the S 2p spectrum can be deconvoluted into two peaks located at 162.68 and 163.73 eV, which are attributed to the S 2p3/2 and S 2p1/2 of the S2- state in the MoS2 nanoparticles, respectively.33
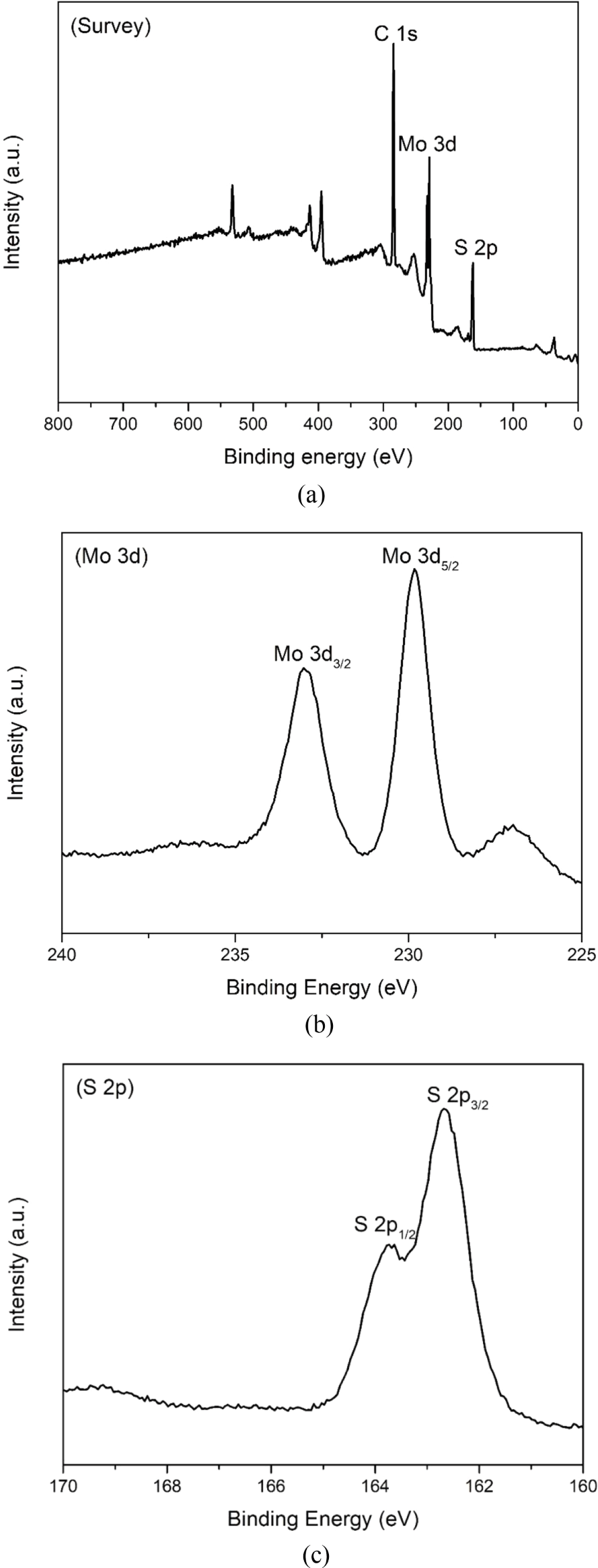
The morphological and structural information of the products was determined using SEM and TEM images. As can be seen in Figure 5, the synthesized MoS2 nanoparticles were connected to each other by the aggregation of irregular-shaped grains. The size of MoS2 is between 50 and 100 nm. In Figure 6, the TEM image of synthesized MoS2 nanoparticles was shown to have a rag structure, and several MoS2 layers were disorderly folded in the rag structure.
The sonocatalytic Orange II degradation experiments were performed using the BBD. The experimental results are summarized in Table 2; the Orange II degradation efficiency ranged from 40.58 to 80.35%. Based on the results in Table 2, the quadratic polynomial regression equation showing the dependence of the Orange II degradation efficiency on the three independent variables, namely, sonocatalyst dosage, dye concentration, and ultrasound treatment time, can be expressed as follows:
To verify the fit of the model, analysis of variance (ANOVA) for the three independent variables was conducted on the basis of the experimental data. The ANOVA results for dye degradation are summarized in Table 3. For this study, high F-value (82.52 and low p-value (<0.0001) indicated that the model was significant. The p-values of the main variables were <0.0001, demonstrating the statistical significance of each variable for the sonocatalytic Orange II degradation. An analysis for lack of fit gives an F-value of 1.69 and a p-value of 0.3048 (>0.05), showing that lack of fit is insignificant.34 Therefore, the quadratic regression model fits well with the obtained data and effectively predicts the experimental results. The determination coefficient (R2), adjusted determination coefficient (Radj2), and predicted determination coefficient (Rpred2) for the regression equation are 0.9907, 0.9787, and 0.9100, respectively. The R2 and Radj2 values were close to 1, indicating a strong correlation between the experimental and predicted values. The difference of Radj2 and Rpred2 is less than 0.2, indicating that Rpred2 is in reasonable agreement with Radj2.26 Based on the results in Figure 7, the experimental and predicted values showed good agreement between the experimental results, and the responses were well predicted by the second-order polynomial model.
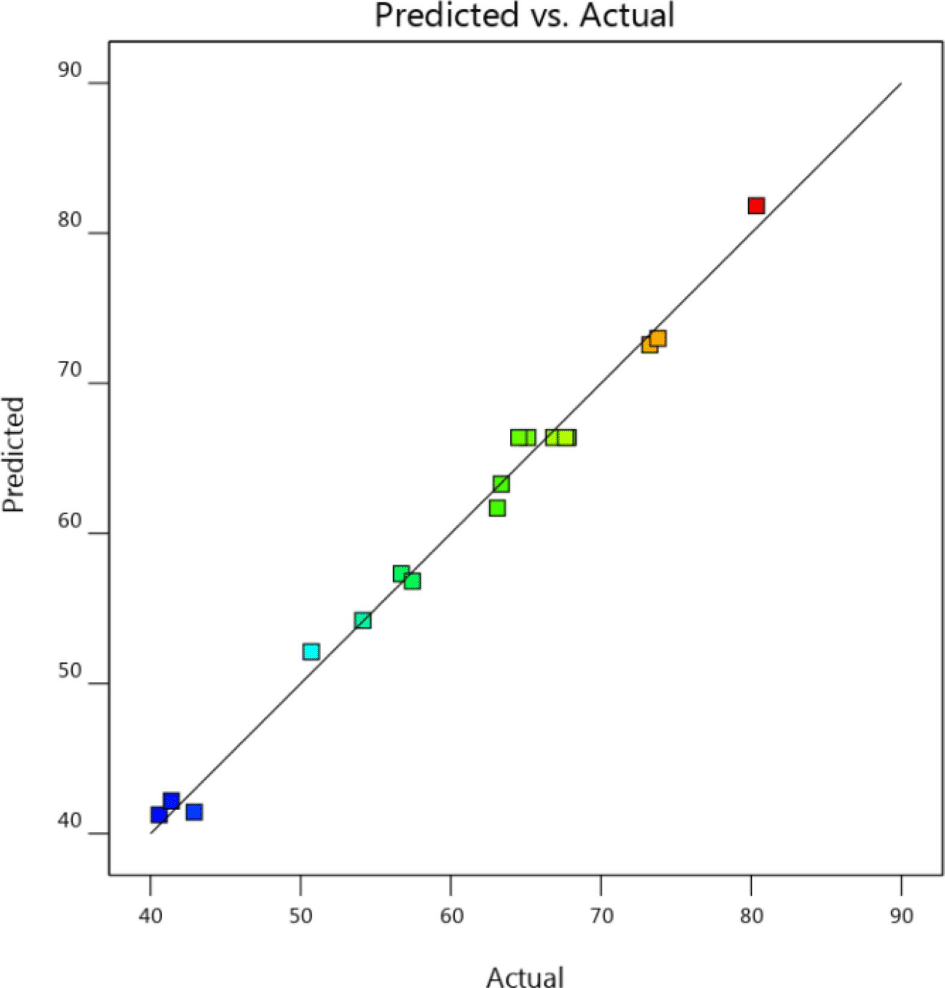
A second-order polynomial equation reveal the type of interaction between two variables by fixing a single variable. This interaction can be reflected more visually by three-dimensional response surface plots and contour plots, which show the significance of the interaction between the two variables.
Figure 8(a) and (b) show the interaction between the initial Orange II concentration and sonocatalyst dosage at a constant ultrasound treatment time. The dye degradation efficiency was improved to some extent by increasing the sonocatalyst dosage. However, the dye degradation efficiency decreased rapidly with an increase in the initial Orange II concentration. This is because at a high initial dye concentration, more dye molecules are adsorbed onto the surface of the sonocatalyst, which leads to a substantial weakening of the effect of sonocatalysis. The lowest degradation efficiency of 40.58% was obtained at a dye concentration of 15 mg/L and a sonocatalyst dosage of 0.2 g/L.
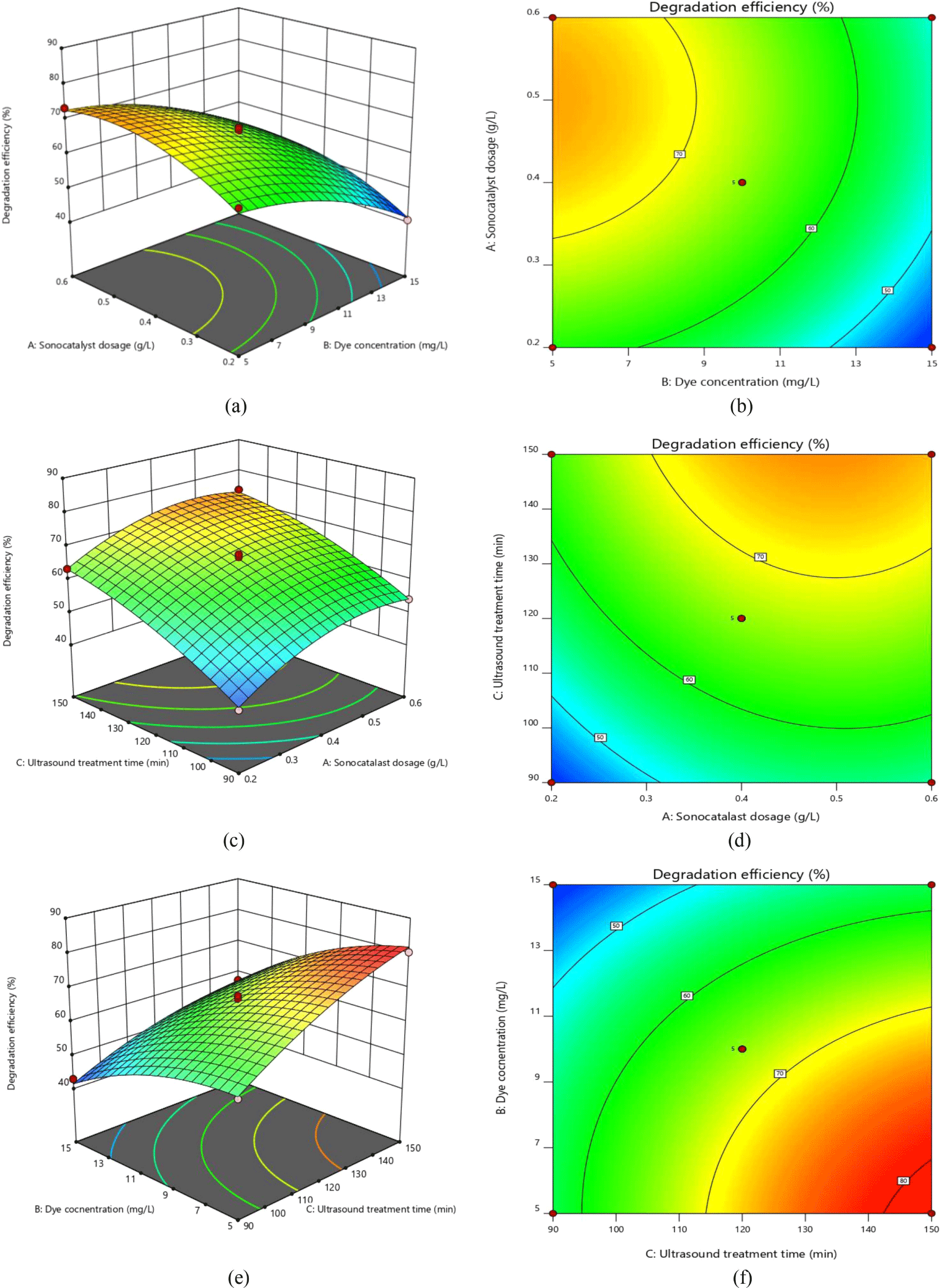
Figure 8(c) and (d) represent the effect of the sonocatalyst dosage and ultrasound treatment time on the dye degradation efficiency at an initial dye concentration of 10 mg/L. It can be clearly seen that an increase in the ultrasound treatment time plays a crucial role in improving the Orange II degradation efficiency. In addition, we found that the magnitude of the change in degradation efficiency caused by an increase in sonocatalyst dosage from 0.4 to 0.6 g/L was lower than that caused by an increase from 0.2 to 0.4 g/L. This may be due to the addition of excessive sonocatalyst, which reduces the dispersion in the system and adversely affects the availability of surface active sites due to local aggregation of the sonocatalyst.
Figure 8(e) and (f) show the effects of the ultrasound treatment time and initial Orange II concentration on the dye degradation efficiency for a fixed dosage of sonocatalyst. With increasing ultrasound treatment time, the degree of ultrasonic cavitation is enhanced, which resulted in the involvement of more reactive radicals in the oxidation of the dye. In this set of experiments, the highest dye degradation efficiency was obtained at an ultrasound treatment time of 150 min, an initial dye concentration of 5 mg/L, and a sonocatalyst dosage of 0.4 g/L. The maximum Orange II degradation efficiency was 80.35%.
Optimizing the numerical values and determining the operational parameters to achieve maximum degradation efficiency were the primary goals of the experimental design. In this study, the experimental conditions were limited to a sonocatalyst dosage of 0.2-0.6 g/L, an Orange II concentration of 5 to 15 mg/L, and ultrasound treatment time of 90-150 min. The operational parameters were optimized to be sonocatalyst dosage of 0.49 g/L, an Orange II dye concentration of 5 mg/L, and an ultrasound treatment time of 150 min by using the optimization function in Design Expert 12. The optimal dye degradation efficiency was predicted to be 82.93% (Figure 9). The error between the model prediction and experimental values was found to be ~3%, demonstrating the accuracy and reliability of the model optimization applied in the sonocatalytic Orange II degradation experiments.
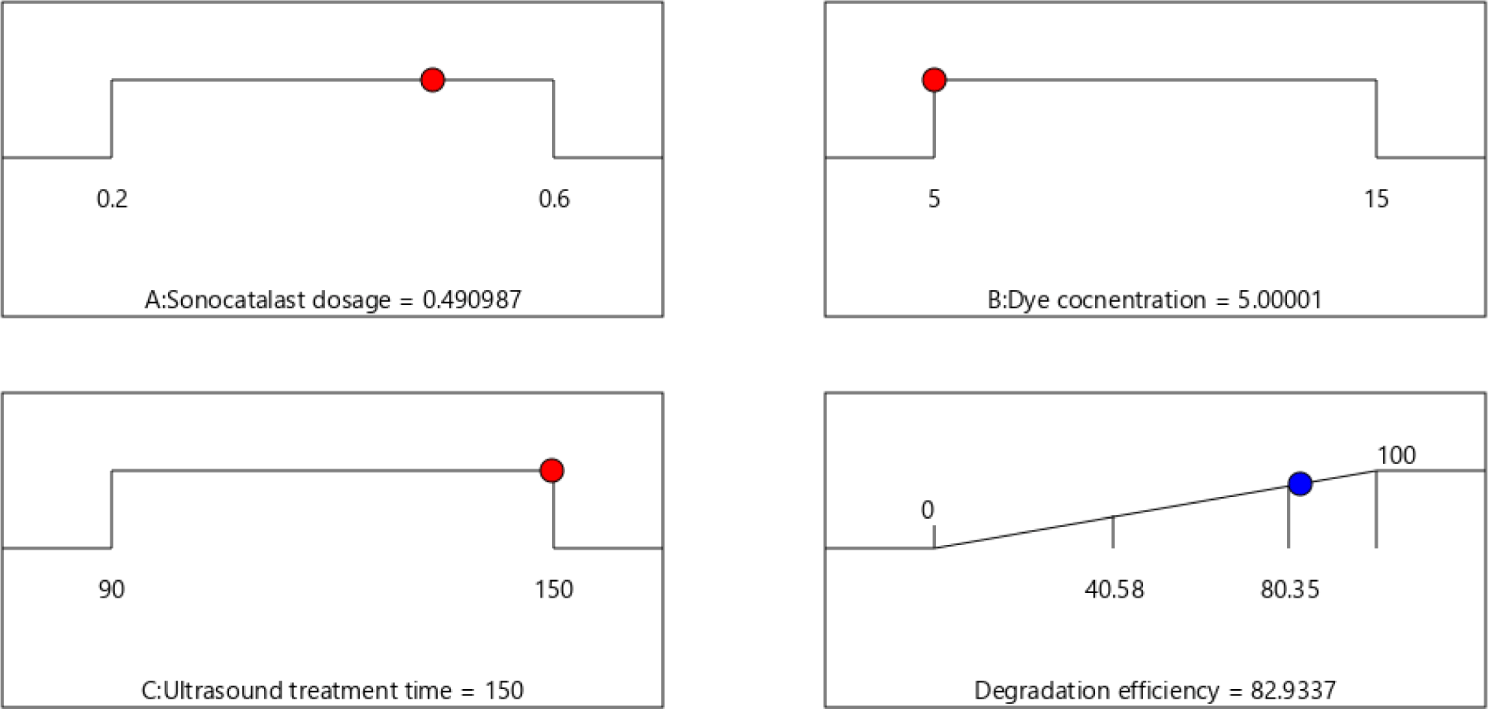
Based on the above experimental results under the optimized conditions, the kinetics of the Orange II degradation on the as-synthesized MoS2 serving as a sonocatalyst was investigated using the Langmuir–Hinshelwood model, which has been widely applied as a model for pseudo-first-order kinetic processes.29,35
The kinetic equation is as follows:
where C0 is the Orange II concentration after adsorption, Ct is the dye concentration at reaction time t, and k is the rate constant of sonocatalytic degradation. Figure 10 shows a plot of ln (Ct/C0) versus the ultrasonic treatment time under the optimized conditions. The rate constant was determined to be 0.0124 min-1. Moreover, the square of the linear correlation coefficient (R2) was > 0.99, indicating that the sonocatalytic Orange II degradation on the MoS2 nanoparticles fit well with the pseudo-first-order model.
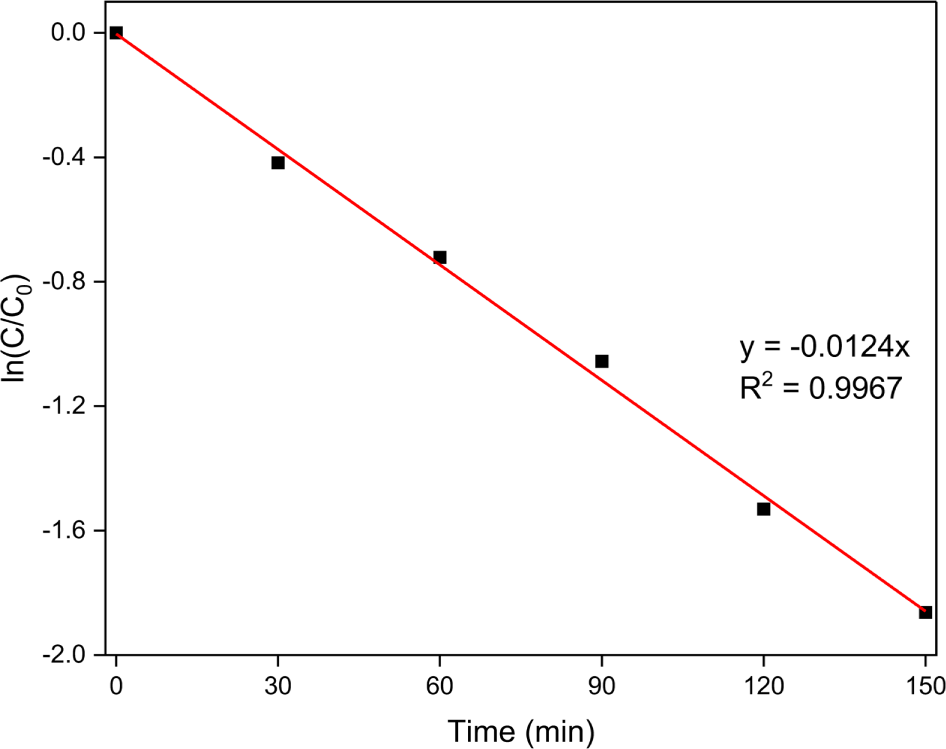
Conclusions
MoS2 nanoparticles were successfully synthesized using a simple hydrothermal and calcination method. XRD, Raman spectroscopy, UV-vis spectroscopy, XPS, SEM, and TEM were used to characterize the as-synthesized MoS2 nanoparticles. The sonocatalytic activity of the samples toward Orange II degradation were evaluated.
The RSM based on BBD approach was utilized to explore the effects of the operational parameters on Orange II dye removal.
The ANOVA results demonstrated that the regression model is highly reliable in predicting the Orange II degradation effi-ciency. The optimal degradation efficiency (82.93 %) was achieved at a sonocatalyst dosage of 0.49 g/L, an Orange II dye concentration of 5 mg/L, and an ultrasound treatment time of 150 min. Moreover, the results of the kinetics study indicated that the sonocatalytic Orange II degradation on the MoS2 nanoparticles fit well with the pseudo-first-order model.