Introduction
In recent years, the importance of high gas barrier properties has been recognized in many fields including packaging and coating. There is a demand for high-performance polymeric material with good gas barrier properties for the application and safety in the rubber industries, such as gas seals, high-pressure hoses, and tires. Almost rubber has a molecular structure that is amorphous, so it is good for gas to pass through. Natural rubber is a well-known elastomer that has been widely used in a variety of industrial fields. The structure of NR is shown in Figure 1. Where black indicates where air (gas) escapes. Due to its poor gas barrier properties, attempts have been made to modify natural rubber via various chemical reaction routes. Epoxidized natural rubber (ENR) is a chemically modified natural rubber, where some of the epoxy groups have been distributed randomly along the natural rubber chain. Commercially available epoxidized natural rubbers that contain 25 and 50 mol% of epoxy contents are referred to as ENR-25 and ENR-50, respectively. ENR has good oil resistance and improved rolling resistance and especially possesses high gas barrier property compared to NR as shown in Figure 2. These properties characterizations are beneficial to optimizing the design of tires and other rubber products. But little effort has been made to understand the mechanism for high gas barrier property of ENR.
In silico (via computational simulation) research on permeability properties of small molecules in polymeric materials is essential to economically develop high-barrier materials. In order to understand the gas transport mechanisms in various polymers of interest, it is useful to associate the chemical composition of the polymer and its morphology with the sorption-diffusion model of penetrants within it. In this regard, molecular simulations have significant advantages in understanding the phenomena of the sorption-diffusion of penetrants in polymer phases at the molecular level. Molecular simulation can estimate the permeation rate of a penetrant molecule through a polymer matrix from the well-known relation, P = DS, where P is the permeation coefficient, S is the solubility coefficient, and D is the diffusion coefficient of the penetrants.1,2 In this sense, molecular simulation has been extensively used to investigate the diffusion mechanism, solubility, and permeability of small gas molecules in polymer matrices including polyethylene (PE),3,4 poly(4-methyl-2-pentyne) (PMP),4 polydimethylsiloxane (PDMS),4 poly(ether imide) (PEI),5 polystyrene (PS),6 and poly(pyromellitimide-1,4-diphenyl ether) (PMDA-ODA) polyimide (Kapton).7
In this study, we predicted and compared the permeability of NR, ENR, and NR/ENR blend (composition ratio of 50 to 50 in NR/ENR). Firstly we calculated the diffusion coefficients of oxygen in each material by the all-atomistic molecular dynamics (MD) simulation. And then, calculations of the solubility coefficients of oxygen were made by the test-particle-insertion method with excluded volume map sampling (EVMS).8 As described above. The permeation coefficients of oxygen in amorphous NR, ENR, and NR/ENR blend were calculated from the diffusion coefficients and solubility coefficients. A cluster analysis of the free volume of theirs was also performed.
Computational Details
In this study, the monomer modeler, polymer modeler, COGNAC modeler and VSOP of J-OCTA software9 developed by JSOL Corporation were used.
Our gas permeability analysis was carried out according to the workflow shown in Figure 3.
The general AMBER force field (GAFF)10 was used for the molecular dynamics (MD) simulation and the restrained electrostatic potential (RESP) approach at the B3LYP/6-31G level was employed to assign partial charges.
All-atomistic model consisting of NR, ENR-50, and their blend (composition ratio of 50 to 50 in NR/ENR-50 blend) was built in this study. Oxygen was chosen as a penetrant. Figure 4(a) and Figure 4(b) show the structures of isoprene and epoxidized isoprene repeat unit constructed with the monomer modeler, respectively. The NR and ENR-50 polymer chain consisting of 60 repeat units, as shown in Figure 5, was built with polymer modeler. As shown in Figure 6, the periodic cells containing 10 polymer chains were constructed with COGNAC modeler. For a blend of NR and ENR-50, the cell was composed of 5 chains of NR and 5 chains of ENR, namely NR/ENR blend with composition ratio of 50 to 50 percentile. To obtain the equilibrated density, the molecular dynamics (MD) equilibration run on each polymer matrix was performed in NPT ensemble with a pressure of 0.1 MPa and temperature of 300 °K. In the NPT ensemble, the number of atoms (N), the pressure (P), and the temperature (T) of the system were kept constant. The temperature was controlled by the Nose-Hoover method, while the pressure was controlled by the Andersen method.
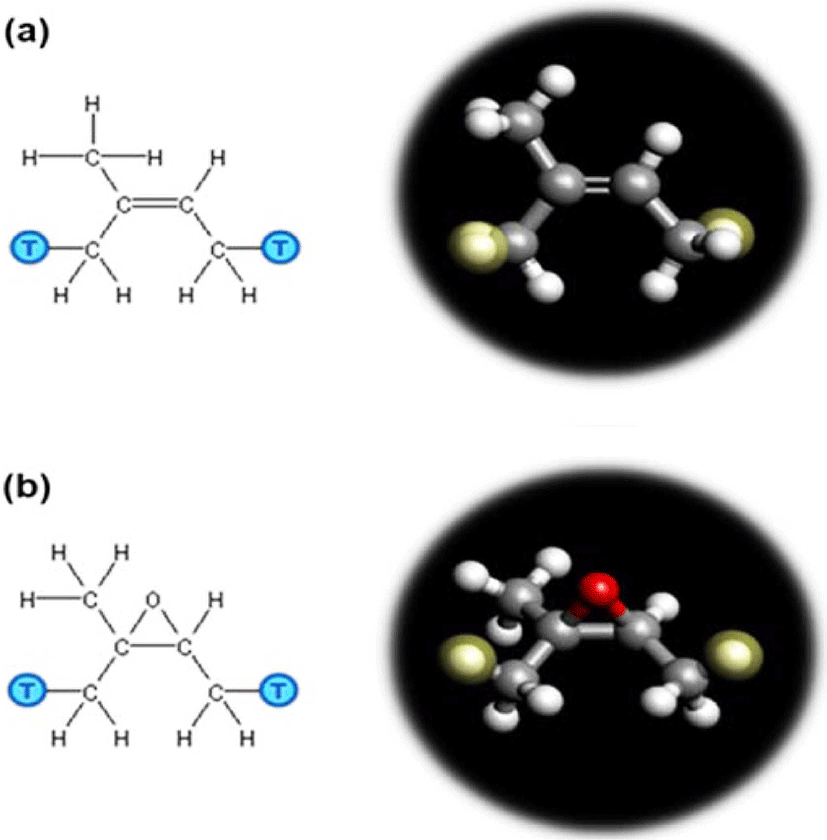
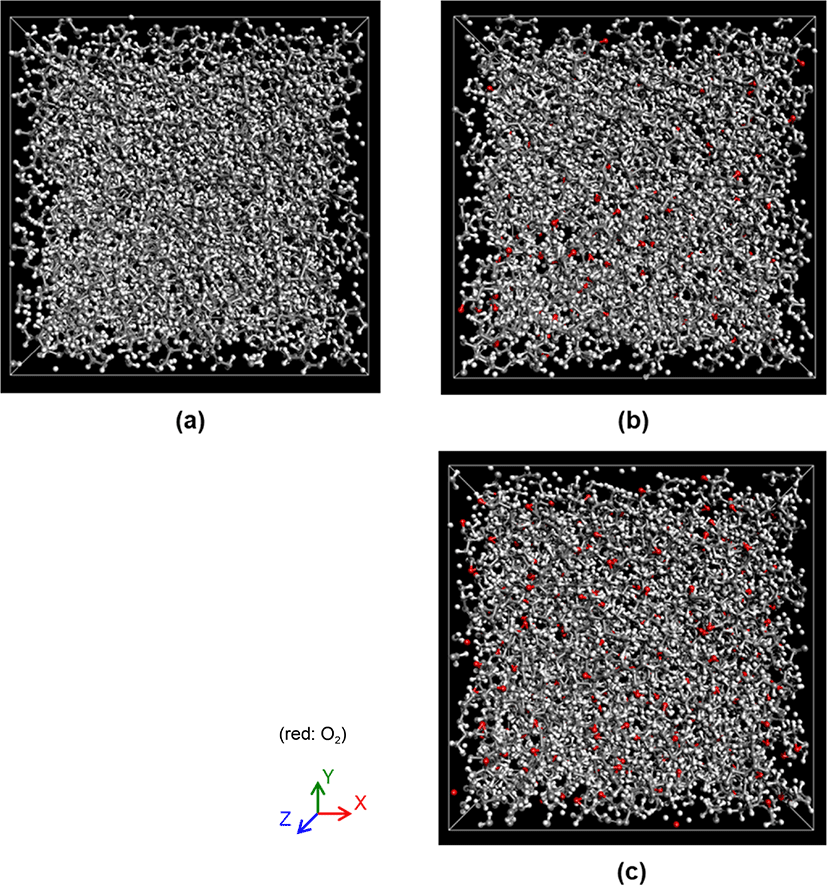
Eight oxygen molecules were inserted at a random location in each of the polymer matrix. In order to investigate the selfdiffusion behavior of oxygen molecules in the polymer matrix, MD simulations were performed in NVT ensemble with temperature of 300°K. In the NVT ensemble, the number of atoms (N), the volume (V), and the temperature (T) of the system were kept constant. The temperature was controlled by the Nose-Hoover method.
The diffusion coefficients, D, were calculated by mean square displacement (MSD) of the center of mass for oxygen by using the Einstein equation,
Where r is the position of oxygen molecules and t is the time. The diffusion coefficients could be calculated by the slope of mean squared displacement (MSD).
The free volumes of the polymer matrices were determined by the test-particle-insertion method with excluded volume map sampling (EVMS).8 A hypothetical atom with the size σ = 2.75 Å was adopted.11 The solubility coefficients, S, were calculated by estimating the excess chemical potential caused by inserting oxygen molecules into the polymer matrix.2
The permeation coefficients, P, of oxygen in the polymer matrix were calculated by multiplying the diffusion coefficients by the solubility coefficients.
Results and Discussion
To evaluate the diffusion coefficient, D, of the oxygen molecules in NR, ENR-50, and NR/ENR-50 blend, we calculated the mean square displacements (MSD) from the 2 nano-second of trajectories of eight oxygen molecules in NR, ENR-50, and NR/ENR-50 blend. Figure 7 shows the mean square displacements (MSD). The calculated values of the diffusion coefficient, D, in NR, ENR-50, and NR/ENR blend are 2.01×10−6, 4.30×10−7, and 8.63×10−7, respectively. The diffusion coefficient, D, of oxygen in ENR-50 has significantly lower than in NR. It can be implied that oxygen diffusion in ENR-50 is much slower than NR. For a NR/ENR-50 blend, the value of the diffusion coefficient, D, is more than one order of magnitude (> 10−1) different from that of NR.
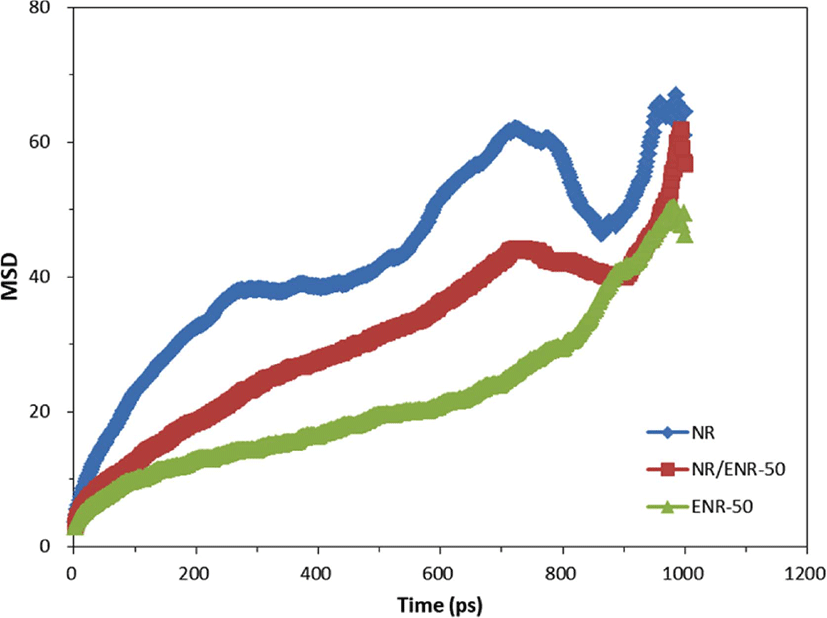
To better understand the difference in the diffusion behaviors of oxygen molecules among NR, ENR-50 and NR/ENR-50 blend, we performed an analysis of the free volume in those materials using a hypothetical atom (diameter 2.75 Å) as a probe. The size of the atom is smaller than that of oxygen. Figure 8 shows the free volume clusters of NR, ENR-50 and NR/ENR-50 blend, respectively. The free volume clusters are expressed by cyan color. The free volume clusters in NR are larger than those in ENR-50. This is attributed to the large diffusion coefficient of oxygen molecules in NR. For a NR/ENR-50 blend, we observed both large free volume clusters and a locally distributed small free volume clusters in the polymer matrix. According to the principle of likes dissolve likes, it can be seen that the large free volume clusters come from polymer chains of NR, whereas the small free volume clusters arise from polymer chains of ENR. NR/ENR blend with the ratio of 50 to 50 percentile has lower diffusion coefficient compared to natural rubber due to the local hindrance of the motion of oxygen molecules by epoxy functional group on the ENR.
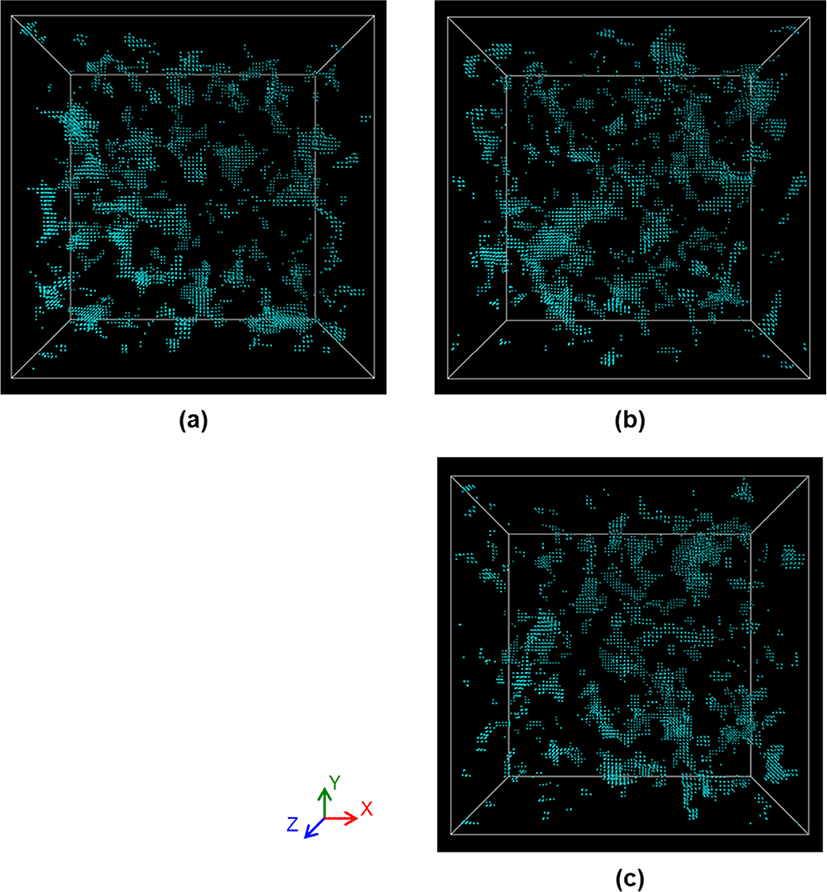
The solubility coefficients, S, were calculated by estimating the excess chemical potential caused by inserting oxygen molecules into NR, ENR-50, and NR/ENR-50 blend are listed in Table 1. The solubility coefficient, S, of oxygen are higher in the order, ENR-50 > NR/ENR-50 blend > NR. It is seen that the content of ENR-50 can increase the amount of oxygen dissolved, thereby reducing the amount of oxygen permeation.
The calculated permeation coefficients, P, of oxygen in NR, ENR-50 and NR/ENR-50 blend are shown in Figure 9. The permeability coefficient, P, of oxygen of ENR-50 is approximately 3 times lower than that of NR, which is in qualitatively good agreement with experiment shown in Figure 2. For NR/ENR-50 blend, the computed value of the permeability coefficient is much lower than that of NR. Our results suggest that permeability of oxygen decreases with volume fraction of ENR-50, whereas the gas barrier property increases with volume fraction. In Figure 9, our trend of evaluated gas permeability are in qualitatively good agreement with experiment for oxygen in NR, ENR-50 and their blends at 23°C.12
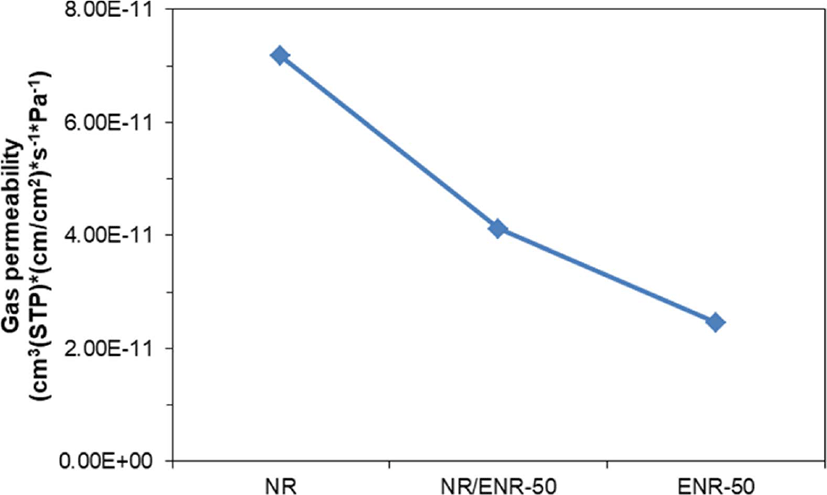
Conclusions
In this work, the molecular simulation has been performed for the evaluation of permeability of oxygen molecules in natural rubber (NR), epoxidized natural rubber (ENR), and NR/ENR (5:5) blend. The main conclusions obtained are as follows:
-
ENR has much lower oxygen diffusivity than that of NR due to its small free volume. When blending NR with ENR, we found that oxygen diffusivity of the blend is lower than that of NR. It can be attributed to the reduction of free volume in the polymer matrix by introducing the ENR.
-
The solubility coefficient of oxygen increases as the content of ENR increases. It can be seen that the content of ENR can increase the amount of oxygen dissolved, thereby reducing the amount of oxygen permeation.
-
NR is more permeable to oxygen but ENR shows maximum oxygen selectivity. In their blend, oxygen permeability decreases with the increasing volume fraction of ENR, whereas the oxygen barrier increases with increasing the volume fraction of ENR, which is in good qualitative agreement with experiment
Our computational simulation on the evaluation of gas permeability can help material scientists to understand mechanism of gas transport in polymeric materials and to efficiently develop the new design of novel polymeric materials with high-barrier properties.