Introduction
Since the first introduction of slide-ring material by Okumura and Ito, a variety of synthesis protocols with reduced fabrication steps and high yield has been suggested.1-4 Polyrotaxane derivatives are mainly composed of three parts; (i) cyclic molecules, (ii) linear molecules, and (iii) bulk end-capping groups (Figure 1).5,6 A bulky end-capping group such as 1-adamantanamine and dinitrobenzene is attached to both ends of a linear molecule inserted inside a cyclic molecule, forming the ring-like supramolecules. Although cyclic molecules are not chemically bound to the linear central chains, the molecules remain stable due to the physical interactions where the mechanically interlocked cyclic molecules can move freely along the linear chain, referred to as mobile interlocked cross-links. Figure-of-eight weak cross-links allow polymer chains to slide through the networks similarly to a pulley system and consequently affect the bulky properties. Binding strength depends on how well the host cyclic molecules are fitted to the linear guest molecules geometrically and on specific local interactions between their surface atoms.
The linear component is usually made from poly(alkylene glycol) such as poly(ethylene glycol) (PEG) and poly(propylene glycol) (PPG), while the cyclic or ring molecules are cyclodextrins (CD), crown ethers, or metal coordination complexes.7,8 Various types of polyrotaxanes can be designable by combining those linear and cyclic molecules. Among them, the PEG-CD supramolecular assemblies are extensively examined as they have good swelling properties and additionally both of these components are biocompatible and biodegradable. The van der Waals interaction between CD and PEG along with the intermolecular hydrogen bonds of CD contributes to the stabilization of the complexes. The number of CD molecules per PEG chains and their distribution affect the properties. When more CDs are threaded onto a given PEG chain, the space for the movement of CDs is restricted and therefore molecular mobility is lowered.
In general, a synthetic procedure of polyrotaxane consists of introducing an end-capping group at the end of both sides and uniformly mixing PEG and cyclodextrin in an aqueous solution. The host cyclodextrins (CDs), an arrangement of glucose units, are environmentally safe, easily available, and economically beneficial. The three most common CDs include alpha(α)-, beta(β)-, and gamma(γ)-CD made up of six, seven, and eight glucose units by alpha-1,4 links.9,10 They have a cylindrical cavity with a height of 7.9 Å and internal diameter of 4.7-5.2 Å for α-CD, 6.0-6.5 Å for β-CD, and 7.5-8.3 Å for γ-CD (Table 1). The cavities possess hydroxyl functionalities on the two rims which make the CD derivatives water soluble. The hydrocarbon and ether moieties located in the inside of the cavity afford the ability to form inclusion complexes with hydrophobic chemicals. For pharmaceutical purposes, the efficiencies of hydrophobic drug such as the stability and bioavailability are improved by increasing dissolution or solubility through the complex formation. When a CD dissolves in water or a polar solvent, the hydrophobic part of the guest species will be entrapped inside of the CD while the hydrophilic parts will stay outside. Formation of the inclusion complex and its stability strongly depends on the appropriate size and shape, interaction of the constituents. For example, the ring of α-CD cannot enter the central polypropylene due to the branches. Therefore, β- or γ-cyclodextrin are suitable. It is often difficult to form a strong bond with the central chain when the CD having large inner diameter is used.
Physical Properties of Polyrotaxane Gels
A gel refers to the polymer networks swollen with a solvent where the networks provide a higher modulus and elasticity, solvent resistance, and improved temperature stability. Polymer networks can be produced either by chemical bonds or weaker physical bonds. The irreversible covalent cross-links are fixed and not distributed evenly throughout the bulky material in the gels. Therefore, mechanical failure easily occurs even upon small deformation. The thermally reversible physical gels are associated with various non-covalent bonds including van der Waals interactions, hydro- gen bonding, or host-guest interaction. Polyrotaxane gels, often called sliding-ring (SR) gels, show high swellability and extensibility where an interlocked crosslinking sites can slide along the polymer backbone.11
The stress-strain curves in Figure 2(a) indicate that the SR gels exhibit lower Young’s moduli and strength compared with those of the fixed cross-link (FC) gels but greater strain-at-break at the same cross-linker concentration.12 For example, the strain of 0.2% SR gels is about 30% higher than the 0.2% FC gels. The modulus and strength values can be tuned by adjusting available sliding distance of cross-links, i.e., the cross-linker concentration. The fracture energies of the SR gels are higher than those of the FC gel. When a single-edge notched specimen of a FC gel is stretched, the polymer strand at the crack tip is finally broken at full stretch, resulting in crack propagation (Figure 2b). Mean- while, the strand length of SR gels at the crack tip is enlarged due to movable cross-links and therefore the crack resistance or fracture energy is improved. The reduced toughness at high moduli is attributable to the decreased network strand length between cross-links. The ratio of the CD covered length of an axis polymer chain, CD coverage, affects the mechanical properties of slide-ring gels. The most polyro- taxane consisting of PEG and CD have 25-30% CD coverage. Many free CDs on the axis polymer chain limit the slipping of the cross-links and cause the failure of the network under the deformation (Figure 3a).13 The slip of CD cross-links over the considerable distance may be of particular importance to obtain gels with a high strength, toughness, and recoverability (Figure 3b).
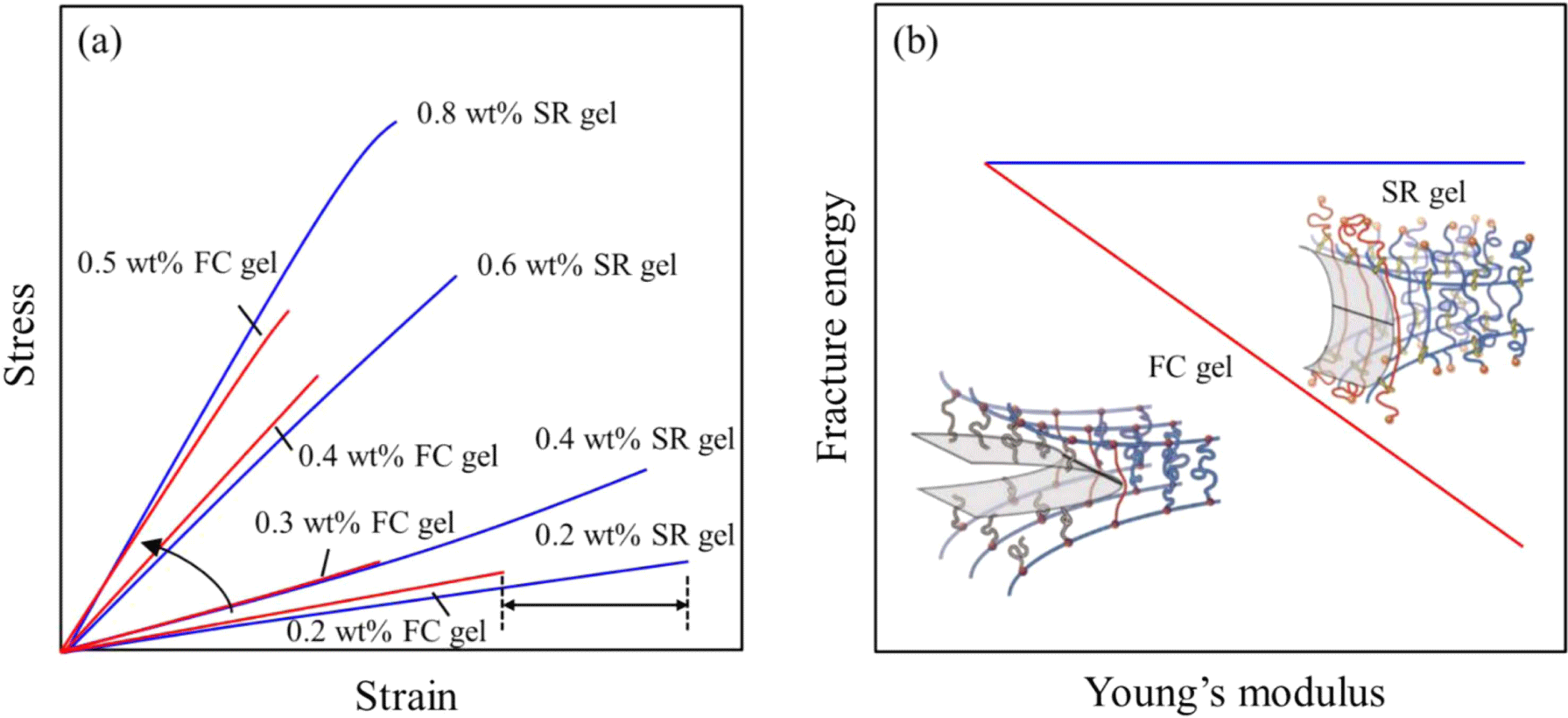
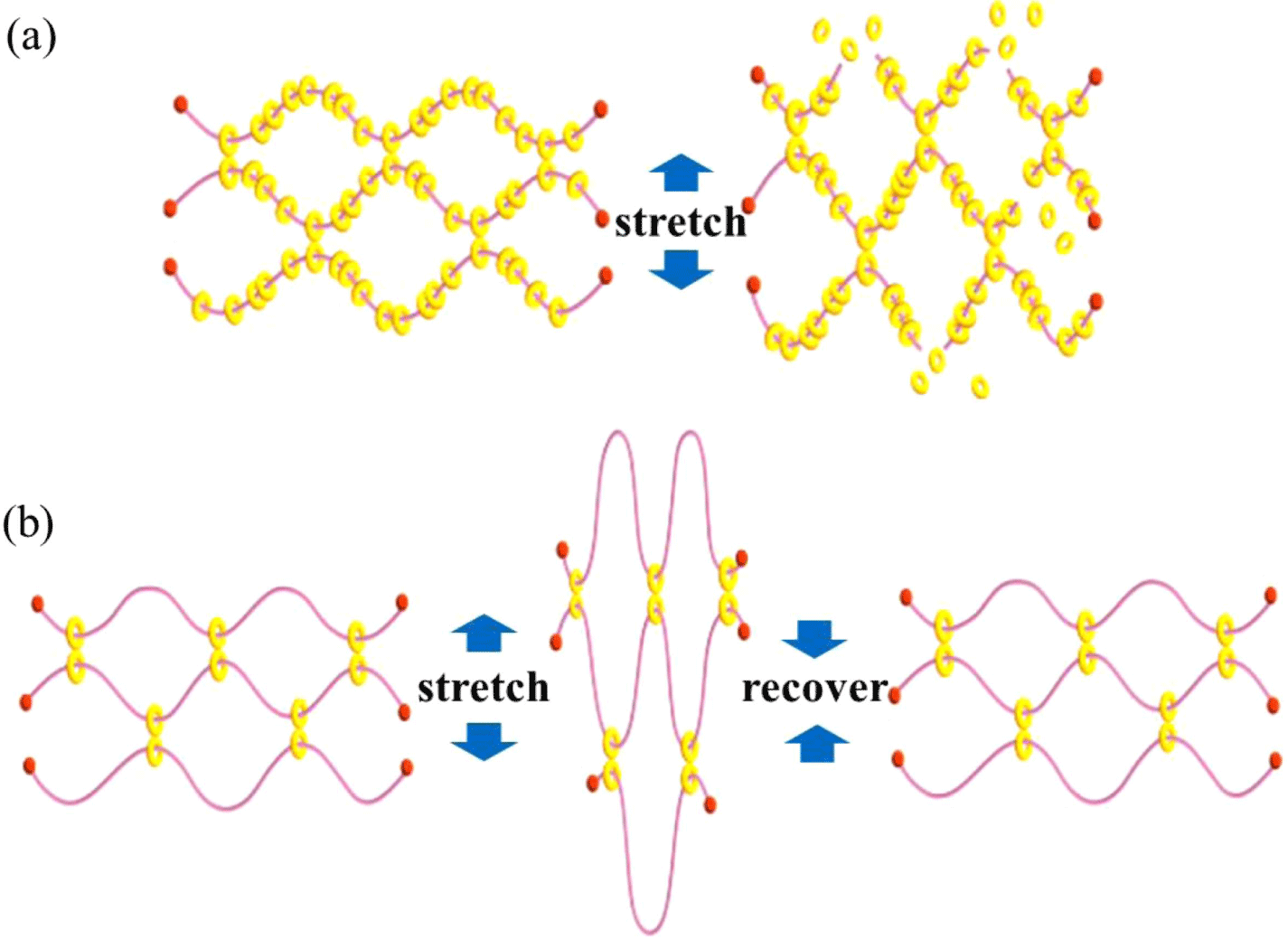
Several hydrogels exhibit a self-healing ability via reversible covalent and non-covalent bonds, but recovery time is still not satisfactory for practical uses. The reversible host-gust interactions with mobile cross-links make the slide-ring gels promising self-healing materials. Compared to conventional hydrogels having a functional group on a polymer side chain, the hydrogels with a functional group modified on polyrotaxane reveal a higher recovery ratio in a relatively short time. Figure 4(a) illustrates the schematic self-healing behavior by host-guest interaction between the adamantane group of polyrotaxane and β-CD containing acrylamide, and corresponding photographs are shown in Figure 4(b).14 It is observed that two gel pieces adhere to form a single gel within a few second after reattachment and moreover the recovery ratio of SR gels is about 20% higher than the conventional adamantane/β-CD gels. Polyrotaxane gels functionalized with phenylboronic acid also shows self-healing ability by dynamic covalent bonds between boronic acid and diol groups (Figure 4c).15 The mobility of the functional units on the end or side chain of the polymer affects the healing time. Self-healing materials could be used in various applications, ranging from scratch-resistant surface coatings of cars and buildings to medical purposes.
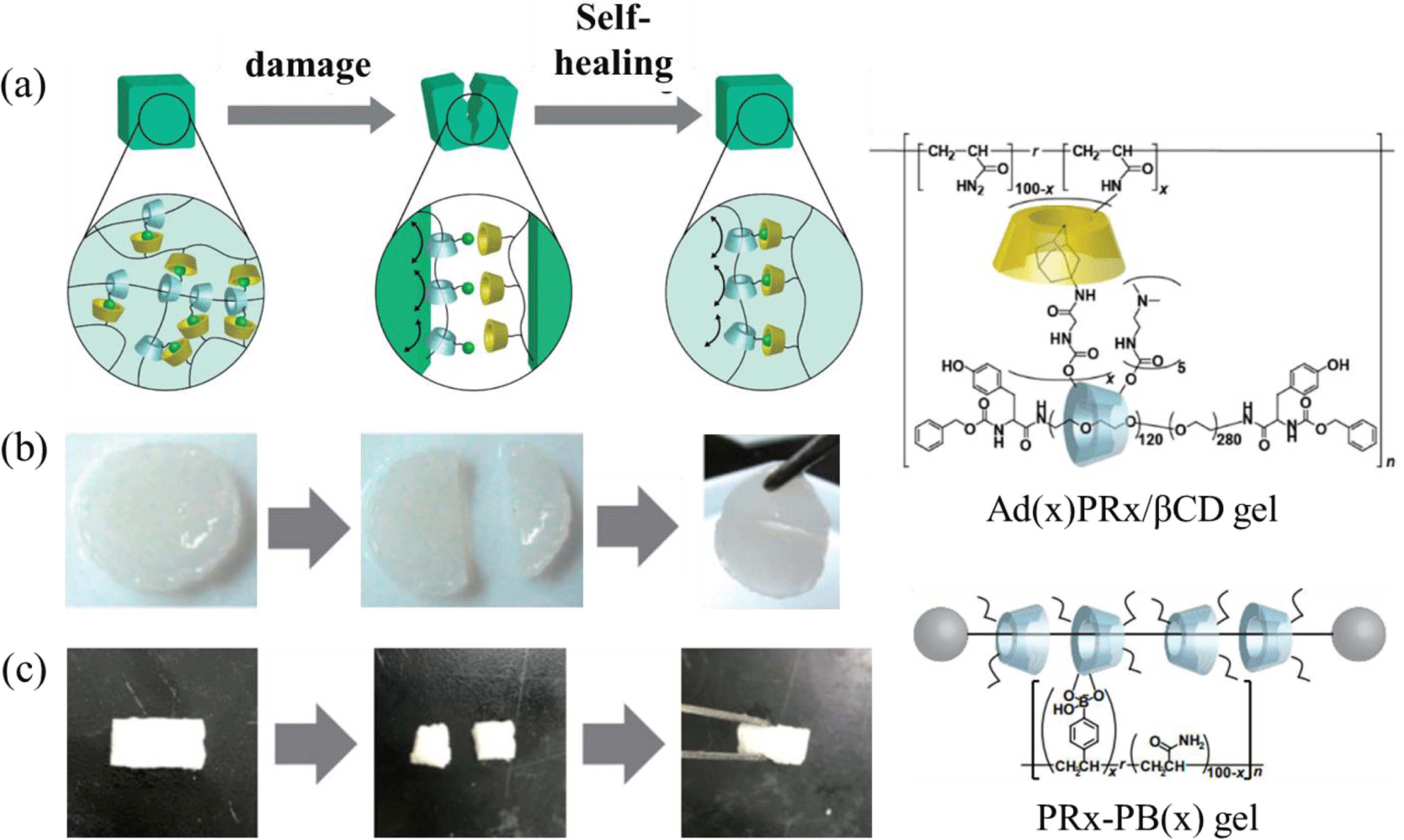
Physical Properties of Polyrotaxane Elastomers
Ideally, elastomer returns to its original shape and length after removing the external tension and has excellent toughness under static or dynamic stresses. Although the physical properties of SR gels with solvent have been widely investigated, there are few studies on SR elastomers because it is more challenging to prepare uniformly cross-linked SR elastomers without the aggregation of ring molecules in a dry state. Cyclodextrin itself or in a complex state tends to aggregate without solvent.16 The polyrotaxane consisting of α-cyclodextrins (CDs) and polyethylene glycol (PEG) forms aggregated crystals of CDs in dry condition. The formation of homogeneous network structures is important to ensure high deformability of elastomers. Solvent-free SR elastomers were successfully prepared by grafting poly-ε-caprolactone (PCL) chains to CDs of polyrotaxanes.17,18 Although the fixed cross-link (FC) and slide-ring (SR) elastomers show similar glass transition temperature (Tg) and elastic mechanical responses at room temperature, the SR elastomers exhibit smaller Young’s moduli at small strains than that of FC elastomers despite the same cross-linking concentration (Figure 5a).19 In the case of FC elastomers, significant strain hardening occurs under large deformation due to stress concentration on polymer networks. In contrast, the stress-extension relation of SR elastomers follows the Neo-Hookean model, i.e., the nonlinear stress-strain behavior under large deformation, without undergoing strain-hardening in a wide range of strains. The slidable cross-links in SR elastomers are responsible for improved flexibility, elasticity, and toughness. No mechanical hysteresis is observed for both the SR and FC elastomers during the loading-unloading cycles (Figure 5b). It should be noted that a simultaneous increase in extensibility, elasticity, and toughness is not achieved using common elastomers.
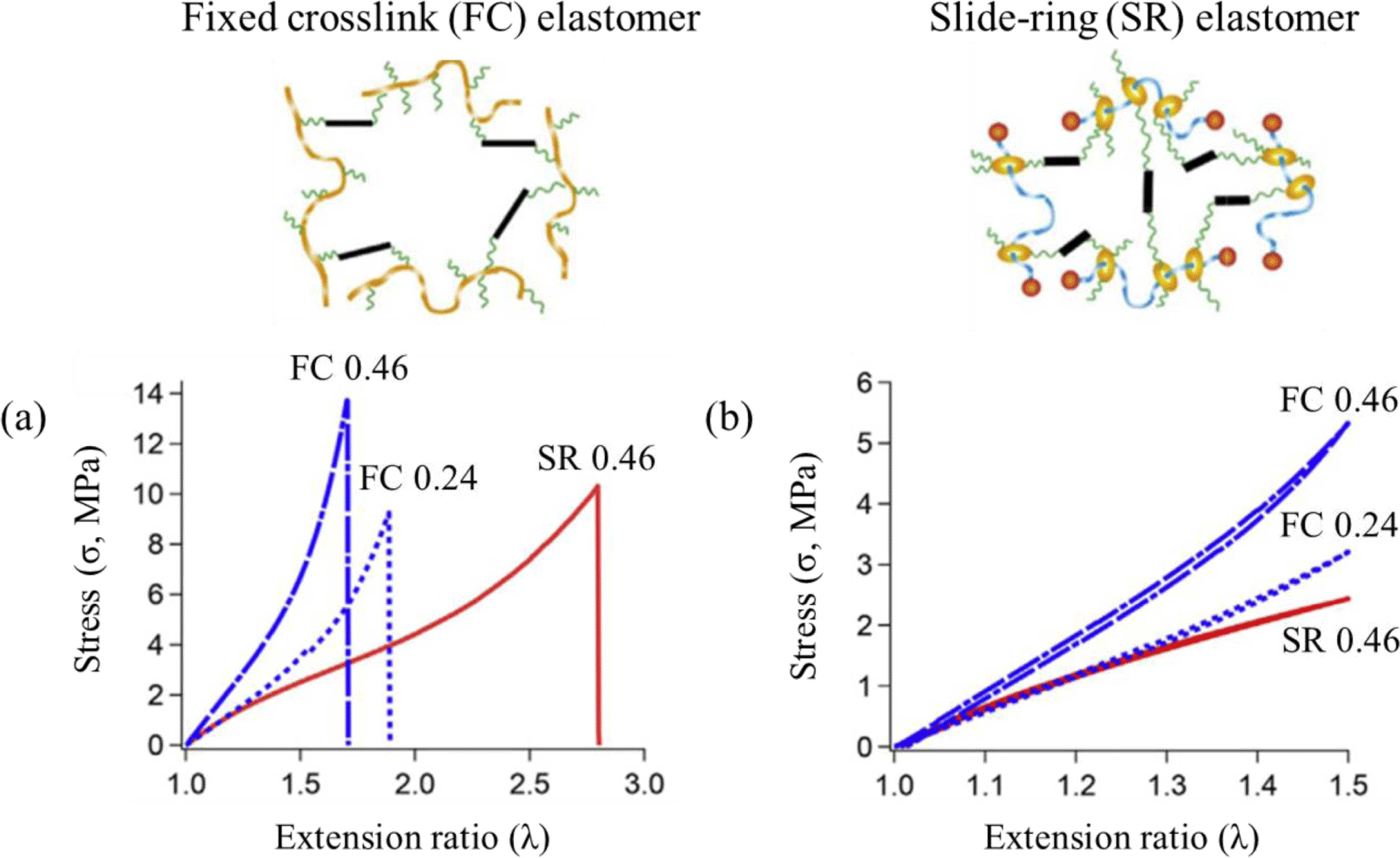
Physical Properties of Polyrotaxane Alloys
The incorporation of polyrotaxane into other polymers is advantageous to modify the mechanical toughness. Polyamide 6 (PA6) is widely used in industries due to its high thermal and mechanical properties. However, PA6 shows low impact properties especially when the compounds have locally high stress and poor dimensional stability. A flexible elastomer is often incorporated into PA6 to provide softness and flexibility. Toray develops flexible and toughened polymers by dispersing polyrotaxane into the nanometer-sized crystals of PA6. The PA6/polyrotaxane nanoalloy exhibits an enhanced bending fatigue limit of about 15-fold compared to the pure PA6 (Figure 6a).20 When 30 wt% glass fiber is reinforced to the alloy, the composites also retain higher breaking elongation and energy absorption more than four times of the PA6-based composites.
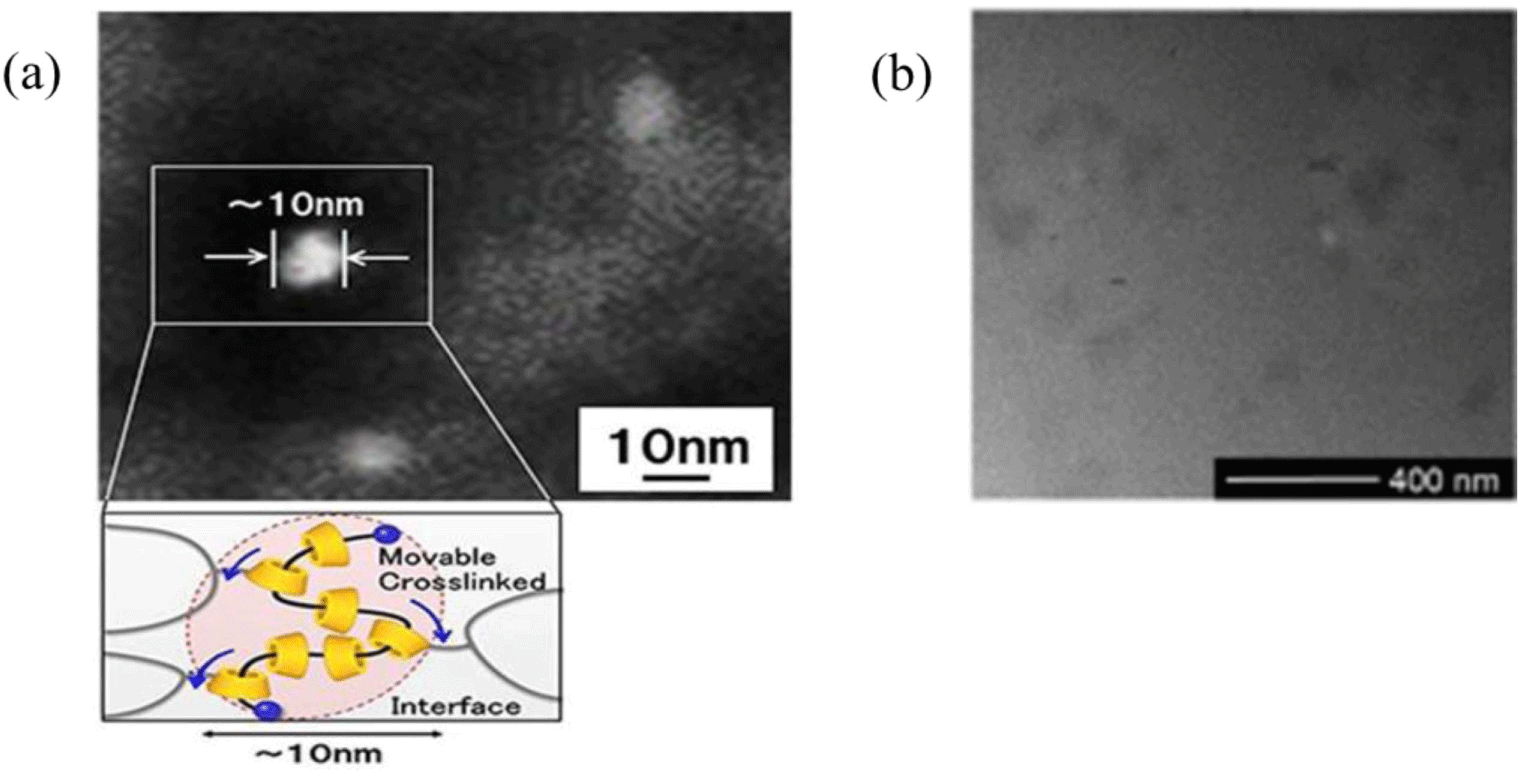
Poly(methyl methacrylate) (PMMA) is an alternative to glass in optical product manufacturing due to its high transparency, hardness, and weather resistance. However, the impact resistance and ductility of PMMA are pretty poor compared to other transparent polymers. Polyrotaxane is used to increase the strain and thus impact strength while maintaining transparency of about 90%. With the aid of a compatibilizer, i.e., styrene/methyl methacrylate/maleic anhydride copolymer, no distinct phase separation is observed, ensuring high transparency (Figure 6b).21 The strain at break of the PMMA mixtures containing 20 wt% polyrotaxane in tensile tests is increased about 2.5 times higher than that of pure PMMA, whereas tensile strength and Young’s modulus are lowered by only 16%. In addition, impact energies are approximately 60% higher due to the chain sliding of polyrotaxane.
Conclusions
Various attractive features of polyrotaxane were reviewed in association with the molecular sliding of cross-links and stress-dissipation ability. Fundamental concepts of the slide ring networks with high toughness and softness applied not only to gels but also to non-solvent elastomers and polymer blends. The sliding of the crosslinking points enlarged the partial strand length between cross-links and led to high toughness and fracture energy of SR gels. Polyrotaxane elastomers were more stretchable and softer than the conventional fixed cross-linked elastomers at the same crosslinking densities without stress-strain hysteresis under the loading-unloading cycle test. The deformation and toughness behaviors of polyrotaxane alloys involving polyamide 6 and polymethyl methacrylate were also briefly addressed in relation to the internal structures and compatibility. Prior to commercial uses of polyrotaxane compounds, it is prerequisite to understand the mechanism of host-guest interaction of selected molecules and precise control of the interfacial compatibility.